Stomach and Duodenum
David K. Magnuson
Marshall Z. Schwartz
Department of Surgery, Case Western Reserve University, Rainbow Babies and Children’s Hospital, Cleveland, Ohio 44106.
Thomas Jefferson University, Philadelphia, Pennsylvania 19107.
Congenital anomalies and acquired abnormalities of the stomach and duodenum are common problems in children. The proper management of these abnormalities, many unique to infants and children, requires an understanding of the anatomy and physiology of the proximal gastrointestinal (GI) tract. The stomach is a complex organ with two distinct physiologic functions: a secretory function effected by the cells of the epithelial lining, and a storage, mixing, and propulsive function effected by the three muscular layers of the gastric wall. These functions are regulated by a complex variety of stimulatory and inhibitory feedback mechanisms that employ neurocrine, endocrine, and paracrine components. The duodenum produces a large number of peptides, many with unknown physiologic roles, and contains the structures that convey bile and pancreatic secretions to the intestinal lumen for mixing with the gastric effluent. Although anatomically, histologically, and functionally distinct, the stomach and duodenum are often considered together because of their proximity and their many interrelated physiologic properties and pathophysiologic responses to disease and stress. This chapter reviews the developmental anatomy of the stomach and duodenum, examines the physiology and pathophysiology of gastric secretory and motor activity, and discusses the treatment of both congenital and acquired gastroduodenal abnormalities in children.
ANATOMY AND PHYSIOLOGY
Developmental Gross Anatomy
The primordial digestive tube forms from the lateral and craniocaudad folding of the embryo during gestational weeks 3 and 4. During this process, the germinal endodermal layer forms the interior of the tube and is surrounded by splanchnic mesoderm. Differentiation of endodermal precursors into surface and glandular epithelium, and of mesodermal precursors into smooth muscle and peritoneal attachments, occurs over the next 6 to 8 weeks. Development of the specialized neuroendocrine cell population of the stomach occurs at about the same time.
Grossly, the stomach begins as a dilation of the foregut, which occurs at about 5 weeks’ gestation. Both the stomach and duodenum are suspended between the posterior and anterior body walls by a dorsal and ventral mesentery. During gestational weeks 6 to 10, the stomach undergoes rotation in two planes. A 90-degree rotation occurs around the longitudinal axis in the clockwise direction when viewed from a craniocaudad perspective, and a lessor rotation around the anteroposterior axis occurs in a clockwise fashion when viewed from the front. Differential growth of the ventral wall of the stomach occurs concurrently. These processes result in the following developmental relations: the greater curvature of the stomach migrates inferiorly and to the left of midline, the gastroesophageal junction is placed superior and to the left, the pylorus moves inferiorly and to the right of midline, the dorsal mesogastrium becomes the gastrosplenic ligament and greater omentum and forms the anatomic boundary of the lessor sac, and the ventral mesogastrium becomes the gastrohepatic ligament. Rotation of the vagal trunks results in the left vagus innervating the anterior gastric wall and liver, and the right vagus innervating the posterior gastric wall, small intestine, and retroperitoneum.
The shape of the stomach and the various epithelial cell types that constitute its mucosal lining create several histologically and functionally distinct zones: the cardia, which surrounds the gastroesophageal junction; the fundus, which projects cephalad from the gastroesophageal junction; the corpus, or body, which represents the largest portion of the gastric reservoir; and the antrum, which describes that portion of the stomach immediately preceding the pylorus (Fig. 72-1). The outer longitudinal and intermediate circular muscle layers (which are concentrated on the greater and lesser curvatures) and the inner oblique layer (concentrated over the anterior and posterior surfaces) compose the three muscular layers of the gastric wall. The gastric wall in the neonate is extremely thin initially, but grows rapidly in the postnatal period in response to the propulsive work associated with enteral feeding.
The gastric blood supply is redundant and derived principally from branches of the celiac axis. The left gastric artery supplies the cardia and proximal lesser curvature; the splenic artery gives off short gastric arteries to the fundus and the left gastroepiploic artery to the proximal greater curvature; the common hepatic artery gives off both the gastroduodenal artery, from which derives the right gastroepiploic artery to the distal greater curvature, and the right gastric artery to the pylorus and distal lesser curvature. Venous return is to the portal vein through the splenic, left gastric (coronary), and superior mesenteric veins.
The duodenum occupies a section of the primitive digestive tube that spans the transition from foregut to midgut. The foregut is proximal to the liver bud and subserved by branches of the celiac trunk, whereas the midgut is distal to the liver bud and subserved by the superior mesenteric artery. The duodenum also undergoes a rotational process. The proximal duodenum is drawn superiorly and to the right during anteroposterior rotation of the stomach, whereas the distal duodenum is drawn leftward by the counterclockwise rotation of the midgut as it returns to the abdomen from the umbilical stalk. The net result of these movements is the C-loop configuration of the normal duodenum. Superimposed on these rotational events is a shortening of the dorsal mesoduodenum, which fixes the duodenum in a retroperitoneal location.
During the sixth to tenth weeks of gestation, the proximal duodenum (along with the esophagus and rectum) undergoes a process of rapid epithelial proliferation that obliterates the hollow lumen, converting the duodenum into a solid, cordlike structure. Vacuoles appear in this homogenous epithelial interior after 8 weeks’ gestation, and gradual recanalization occurs as these vacuoles coalesce into larger cavities. The distal duodenum is believed not to pass through a solid phase.
The duodenum is divided into four portions corresponding to the curvatures of the C loop. A consistent landmark is the ampulla of Vater, which is located medially in the second portion and represents the confluence of the common bile and pancreatic ducts and their entry into the duodenum. The duodenal blood supply is derived from the celiac axis through superior pancreaticoduodenal branches of the gastroduodenal artery, and the superior mesenteric artery through inferior pancreaticoduodenal branches. Venous drainage is through corresponding branches to the portal vein.
During this same period, the pancreas develops from separate dorsal and ventral anlagen. The ventral anlage arises to the right of midline, and as the intestinal tract undergoes rotation, this structure also rotates posterior to the duodenum and fuses with the dorsal anlage to the left of the duodenal C loop. The two separate ductal systems fuse, forming the main pancreatic duct of Wirsung and a minor accessory duct of Santorini. Abnormalities of pancreatic migration and fusion result in ductal anomalies (pancreas divisum) and parenchymal anomalies (annular pancreas). The frequent coexistence of annular pancreas with congenital duodenal obstruction suggests that the anatomic development of these structures is closely interrelated.
Developmental Neuroanatomy
The stomach is richly innervated by all three components of the autonomic nervous system—sympathetic, parasympathetic, and enteric—although functionally the latter two predominate. Sympathetic fibers in which the postganglionic neurotransmitter is primarily norepinephrine are generally inhibitory to GI function, whereas parasympathetic pathways mediated by acetylcholine are generally stimulatory. In contrast, the enteric nervous system (ENS) is characterized by a wider variety of neurotransmitters, including dopamine, somatostatin, vasoactive intestinal peptide (VIP), gastrin-releasing peptide, ghrelin, and cholecystokinin (CCK), and a wider variety of effector and regulatory functions. Many of the peptides used by the ENS as neurocrine mediators are also elaborated by enteroendocrine cells as endocrine and paracrine mediators.
The sympathetic and parasympathetic innervation of the stomach is well defined. Sympathetic innervation originates from cell bodies within the thoracic spinal cord and extends through presynaptic fibers in the greater splanchnic nerve to postsynaptic neurons in the celiac ganglion, whose axonal fibers follow blood vessels into the gastroduodenal wall. This arrangement correlates well with the principal sympathetic function of vasomotor control. Sympathetic innervation of the muscular layers is sparse and is concentrated chiefly in the pyloric sphincter. Parasympathetic presynaptic nerves originate in the brainstem and follow the vagus nerves to the stomach. Interestingly, efferent impulses constitute only about 20% of the total neural traffic in the vagus nerves. The entire gastric wall is richly innervated by terminal vagal fibers branching off the left (anterior) and right (posterior) vagal trunks as they give off their hepatic and celiac divisions, respectively. These presynaptic fibers synapse with intramural ganglia and also integrate at this level with ENS ganglion cells. Parasympathetic innervation mediates predominantly prosecretory and prokinetic functions.
The ENS is the largest and most complex compartment of the autonomic nervous system and comprises more than 108 resident neurons within the walls of the GI tract. It is distinguished from the sympathetic and parasympathetic systems by its anatomic separation from the central nervous system (CNS). It has long been recognized that the ENS functions more or less independently, but receives modulatory input from the central autonomic system, mostly by way of the vagus (parasympathetic) nerves. When one considers that the efferent fibers of the vagal trunks at the esophageal hiatus number only about 2,000, it is clear that most of the ENS neurons receive stimulatory and inhibitory inputs from other ENS neurons (1). Thus, the inherent myoelectric activity of GI smooth muscle is under both intrinsic (ENS) and extrinsic (CNS) control.
ENS precursors differentiate from neuroblasts located in the vagal area of the neural crest and migrate with the vagus nerves to the developing stomach and GI tract (2). Once incorporated into the gastric wall, these ENS neurons further differentiate, proliferate, and establish connections to each other, to other autonomic pathways, and to developing gastric secretory and muscle cells. Although the migrating precursors transiently express immunoreactivity for catecholamines, once established within the gastric wall, they no longer express catecholamines. ENS plexuses are found within virtually every histologic layer of the stomach, integrating with ganglia found in the mucosa, the intermyenteric plane, and the subserosa.
Developmental Histology of the Gastric and Duodenal Epithelium
The gastric epithelium is an aggregation of diverse cell populations that are distributed in a regionally specialized manner and that account for the various secretory functions of the stomach. Seen from the luminal surface, the gastric mucosa is a monotonous sheet of mucus-secreting columnar epithelial cells riddled with tiny openings referred to as gastric pits. At the base of these pits are the gastric glands, which are specialized tubular invaginations of the mucosa that contain the effector and regulator cells of gastric secretion. Gastric glands are composed of different cell populations in various regions of the stomach, allowing the stomach to be compartmentalized by histologic and functional characteristics.
The cells that occupy the surface zone throughout the entire stomach secrete mucus, which provides a protective barrier between luminal acid and the gastric wall. These surface mucous cells are simple columnar epithelium with projecting microvilli. Immediately subjacent to the villi are mucous granules, which fuse with the apical cell membrane and deliver mucus by exocytosis. An extensive and active Golgi apparatus and rough endoplasmic reticulum are seen in the cytoplasm. These cells continuously recycle every 72 hours. Mucous neck cells are found deeper in the neck of the gland and have a similar morphology and function. They appear to renew every 7 days.
Parietal cells are perhaps the most functionally unique cells found in the gastric epithelium. They produce both hydrochloric acid (HCl) and intrinsic factor (IF) and are under a complicated system of regulatory controls. In the resting state, these cells contain unusual intracellular smooth membranes termed tubulovesicles. When stimulated, these structures are replaced by extensive intracellular canaliculi that communicate directly with the glandular lumen at the apical interface and that contain numerous microvilli. These microvilli appear to be the site of greatest concentration of the H+-K+-ATPase (proton pump) that drives HCl secretion against a large concentration gradient. Consistent with such an energy-intensive process, these cells contain large mitochondria in a concentration exceeded only by the myocardium. Parietal cells are found throughout the isthmus and neck regions of the glands, predominately in the gastric fundus and body and less often in the proximal antrum. They can be identified in gastric glands as early as gestational week 10.
Chief cells are found exclusively at the base of the gastric glands. They synthesize, store, and secrete pepsinogen, which is hydrolyzed to the active proteolytic enzyme pepsin in the acid environment of the gastric lumen. Pepsinogen is stored in apical zymogen granules in preparation for release by exocytosis. These cells are found principally in the gastric fundus and body, and first appear in the twelfth week of gestation.
Enteroendocrine cells are present throughout the stomach, duodenum, and distal intestine (Fig. 72-2). These cells have in common the ability to internalize certain precursor molecules and produce biologically active amines and peptides by intracellular decarboxylation. They are therefore referred to as amine precursor uptake and decarboxylation cells. Enteroendocrine cells also interact with ENS fibers within the gastric and duodenal walls. Together, the enteroendocrine cells and ENS fibers produce a variety of signaling molecules that integrate and regulate the complex events underlying acid secretion, motility, digestion, and metabolic response (Table 72-1).
Many distinct types of enteroendocrine and neurocrine cells are found in the gastric mucosa. The most common and well-characterized are the G cells, which produce gastrin, and the D cells, which produce somatostatin and amylin. Others produce such diverse amines and peptides as serotonin, histamine, dopamine, vasoactive intestinal peptide (VIP), glucagon, gastrin releasing peptide (GRP), motilin, and ghrelin. Because these substances function in endocrine and paracrine regulation, the cells that produce them tend to have high concentrations of secretory granules at their basolateral (i.e., abluminal) membranes. Microvilli on their apical (luminal) membranes contain the chemoreceptors and pH receptors necessary to sense environmental changes and function in feedback inhibition. Another enteroendocrine cell, the enterochromaffin-like (ECL) cell, produces histamine, perhaps the primary stimulus for acid secretion. Enteroendocrine cells are ubiquitous both within the gastric glands and within the duodenal wall. The most common types, the G and D cells, predominate in the gastric antrum.
![]() FIGURE 72-2. Distribution of selected enteroendocrine and neurocrine (asterisk) cells and their products in the proximal gastrointestinal tract. |
Enteroendocrine cells are among the first to populate the gastric glands, emerging at 8 to 9 weeks’ gestation. They derive from stem cells located within the base of the gastric gland. Certain cells, referred to as A cells, appear to produce glucagon and are only present in fetal and neonatal glands. Such observations, along with the recognition that many GI hormones have trophic effects, have led to the hypothesis that some enteroendocrine cells may participate in the growth and differentiation of the fetal stomach GI tract. Finally, a large number of undifferentiated cells can be seen interspersed with other cell types in the gastric glands. Their role is postulated to be the regeneration of all nonenteroendocrine components of the epithelium after injury. Enteroendocrine regeneration appears to result from type-specific precursors.
TABLE 72-1 Important Intercellular Signaling Molecules in the Stomach and Duodenum. | |||||||||||||||||||||||||||||||||||||||||||||||||||||||||||||||||||||||||||||||||||
---|---|---|---|---|---|---|---|---|---|---|---|---|---|---|---|---|---|---|---|---|---|---|---|---|---|---|---|---|---|---|---|---|---|---|---|---|---|---|---|---|---|---|---|---|---|---|---|---|---|---|---|---|---|---|---|---|---|---|---|---|---|---|---|---|---|---|---|---|---|---|---|---|---|---|---|---|---|---|---|---|---|---|---|
|
Based on the cell types previously described, it is possible to segregate the stomach into three histologically and functionally distinct regions characterized by the presence of one of three specific gland types: cardiac, oxyntic, or pyloric. Cardiac glands are devoid of parietal and chief cells, and occupy a 1- to 2-cm ring around the gastroesophageal junction. Oxyntic glands are the most prevalent; they occupy the fundus and most of the body. These glands contain all the cell types listed earlier, and are especially rich in parietal and chief cells. Pyloric glands, found in the distal 10% of the stomach, have few parietal and no chief cells, but are well endowed with enteroendocrine cells, especially G and D cells.
The duodenal mucosa is also richly populated with enteroendocrine cells. These cells are stimulated by nutritional substrates delivered from the stomach, and they secrete mediators that regulate a variety of digestive processes. Secretin is produced by duodenal S cells in response to luminal acid, stimulating bicarbonate secretion from the pancreas, liver, and duodenal Brunner glands and mucosal cells. CCK is produced by duodenal I cells in response to certain fatty acids and amino acids; it stimulates gallbladder contraction and pancreatic exocrine secretion. Secretin and CCK have also been proposed to have trophic effects on the fetal intestinal tract and pancreas, respectively. Glucagon-like peptides 1 and 2, previously termed enteroglucagons, are produced by L cells in the duodenal and distal gastrointestinal mucosa, and govern such diverse events as insulin secretion and gastric motility (3). A partial list of these cells and their putative functions is found in Table 72-1.
Physiology of Gastric Motor Function
The coordinated muscular activity of the stomach and duodenum, like that of the rest of the GI tract, is the net result of many complex interactions between mural smooth muscle cells and the ENS. The stomach is divided into two distinct functional zones based on marked differences in motor activity. The proximal stomach, including the fundus and proximal third of the body, exhibits the properties of receptive relaxation and accommodation, and serves as a reservoir in which the ingested meal is stored. The ability to distend without a concomitant increase in intraluminal pressure is important during bolus feeding. In addition, the proximal stomach generates slow, sustained, tonic contractions that elevate mean intragastric pressure above duodenal pressure and provide a constant pressure gradient that controls the passage of liquids through the stomach. These properties are under significant CNS modulation by nonadrenergic, noncholinergic vagal fibers. Therefore, vagotomy significantly impairs these functions and causes rapid emptying of liquids. The remainder of the stomach distal to the first third of the body is functionally and physiologically distinct from the proximal stomach. Motor activity in the distal stomach is characterized by spontaneous myocyte membrane depolarizations that result in phasic, directional contractions. These contractions account for the ability of the distal stomach to mix and grind solid food and to empty mixed food particles into the duodenum in a controlled fashion.
A basic property of smooth muscle cells in the distal stomach and duodenum (but not the proximal stomach) is a spontaneous slow depolarization of their membrane potential from a resting level of about -70 mV toward zero. Because myocytes are physiologically linked through gap junctions, the slow wave depolarizations of one cell are communicated to adjacent and more distant cells through serial connections. This leads to a spreading wave of depolarization in which cells with the most frequent spontaneous membrane depolarizations entrain those with lower frequencies. In the stomach, the cell population with the highest rate of spontaneous depolarization, referred to as the gastric pacemaker, is located along the greater curvature at the proximal boundary of the distal zone. Depolarizations spread aborally through the body, antrum, and pylorus without being propagated into the proximal zone. The rhythmic activity of the gastric pacemaker is about 3 to 4 cycles per minute (in the duodenum, which can be considered to be a small intestine pacemaker, slow waves are generated at a rate of about 10 to 12 cycles per minute).
Slow wave depolarizations are electrical events. They are coupled to mechanical contractions only when the depolarizations exceed a threshold potential, at which time a rapid depolarization to 0 mV occurs (termed an action potential) and triggers smooth muscle contraction by a rapid increase in intracellular calcium. When an action potential of sufficient magnitude is generated, a series of rapid action potentials follows before repolarization can occur (termed a spikeburst); this results in a sustained muscular contraction sufficient to elevate intragastric pressure. The underlying organization of slow wave depolarization dispersion ensures contractions are organized in a propulsive or peristaltic fashion. The frequency, direction, and magnitude of muscular contraction is under intrinsic and extrinsic neurocrine controls that alter resting membrane potentials, threshold potentials, and action potentials as a means of regulating the translation of slow wave depolarizations into mechanical contractions.
During the fasting state, gastric myoelectric activity follows a repetitive pattern with a period of about 90 to 120 minutes, called the interdigestive migrating motor (myoelectric) complex (MMC). The MMC can be divided into four phases. Phase I is mechanically silent, without the generation of action potentials or muscular contractions. In phase II, random, low-amplitude contractions occur. Phase III is characterized by regular, intense muscular contractions resulting from the conversion of every slow wave depolarization into an action potential with spikebursts. This phase empties the gastric lumen of all indigestible materials and is responsible for imparting the term housekeeper to describe the MMC. A short phase IV is marked by a gradual return to phase I quiescence.
The fed state occurs when the interdigestive MMC is interrupted by the arrival of ingested food in the stomach. The regular pattern of MMC activity is replaced with forceful, nonpropagated contractions in the distal stomach, coupled with coordinated contractions of the pyloric sphincter, that serve to churn and grind food into small particles. Distal gastric motor activity appears to be controlled by multiple factors: Vagal cholinergic activity, gastrin, GRP, CCK, neurotensin, motilin, and ghrelin stimulate contractions, whereas adrenergic and noncholinergic vagal activity, somatostatin, secretin, VIP, GLP 1 and 2, and GIP inhibit them.
When the average particle size approximates 1 mm in diameter, chyme is allowed to empty into the duodenum. The particulate composition and osmolality of chyme affects the rate of emptying: Carbohydrates empty faster than proteins, which empty faster than fats. Isocaloric amounts of nutrients, however, empty in about equal times. Intraduodenal acid, carbohydrate, and fat stimulate intramural, cholinergic ENS feedback loops that result in pyloric contraction, slowing gastric emptying. This complex set of interactive factors ensures the rate of gastric emptying is adjusted to provide an isocaloric flow of nutrients into the duodenum over time.
Physiology of Gastric Secretion
Production and secretion of HCl by gastric parietal cells is governed by a complex, highly redundant network of stimulatory and feedback controls that involve neurocrine, endocrine, and paracrine pathways. Establishing a dominant or final common pathway has been difficult because the parietal cell and other regulatory cells have been shown to possess cell surface receptors for a wide variety of molecular secretagogues. The parietal cell receives input from cholinergic and noncholinergic nerve terminals of the autonomic nervous systems (sympathetic, parasympathetic, and enteric), from hormones delivered through the microvasculature from distant sources (e.g., gastrin from antral G cells), and from peptide and amine messengers secreted into the local interstitial environment (e.g., histamine from fundic ECL cells). Each cell elaborating these regulatory substances is, in turn, subject to regulatory inputs equally complex.
Functions of acid include initiating and facilitating the hydrolysis of peptide bonds during protein digestion by denaturing ingested proteins and exposing specific amino acid sequences to pepsin, an endopeptidase that requires an acidic environment for activation. Because patients with chronic achlorhydria do not often suffer from malabsorption, the importance of acid-peptic digestion may not be critical. Nevertheless, when acid secretion is normal, it plays an integral role in initiating the digestive process.
An additional function of gastric acid is to create a barrier to the entrance of bacteria into the GI tract. Gastric acidity not only protects the upper aerodigestive tract from potentially pathogenic bacteria found in the lower GI tract, but also insulates the various populations of indigenous bacteria inhabiting the lower tract from constant challenges by new strains of ingested microorganisms. Chronic neutralization of gastric acidity results in bacterial overgrowth, which can lead to nosocomial respiratory tract infections, digestive abnormalities, and possibly generation of carcinogenic nitrosamines.
Gastric secretory function evolves early in development. By 10 weeks’ gestation, parietal and enteroendocrine cells have begun to differentiate, and by 12 to 13 weeks, gastrin, HCl, pepsin, and IF can all be detected. Mucus and bicarbonate secretion commences later, at about the sixteenth week of gestation. Further prenatal growth and development is probably under enteroendocrine control. In the newborn, the gastric luminal pH is neutral, owing to swallowed amniotic fluid. Within the first several hours of life, however, acid secretion is sufficient to reduce the pH to 3.5. Further secretion lowers the pH to 1.0 to 3.0 by 48 hours, with little distinction between basal and stimulated outputs. Thereafter, acidity declines until the third week, when HCl secretion begins to increase again. By the age of 3 to 4 years, acid secretion approximates adult levels. Premature infants have a prolonged period of alkalinity, often extending for several days. As expected, the smallest premature infants display the most prolonged delay in acid secretion.
Control of Acid Secretion
Parietal cells in the fundus and corpus display surface membrane receptors for a variety of substances that stimulate or inhibit production and secretion of HCl. Stimulatory substances and their receptors include histamine (H2 receptor), acetylcholine (M3 receptor), and gastrin (gastrin receptor); inhibitory substances include somatostatin (somatostatin receptor), epidermal growth factor (EGF) receptor, prostaglandin E (PGE) series prostaglandins (EP3 receptor), and possibly adrenergic neurotransmitters (β-adrenergic receptors). Although the heterogeneity of receptors suggests that receptor-mediated acid secretion can be effected through a number of pathways, the observation that competitive inhibition of H2 receptors can abolish acid output in response to all secretagogues strongly supports a central role for histamine as the final common pathway for most acid secretion under physiologic conditions. Gastrin alone is a relatively weak activator of isolated parietal cells in culture (4). The primary function of both acetylcholine and gastrin at the parietal cell membrane is probably to potentiate the effect of histamine because synergistic responses in acid secretion are seen with combinations of histamine and either gastrin or acetylcholine (5).
Although the source of gastric histamine was originally presumed to be the mast cell, gastrin has no demonstrable effect on mast cell histamine secretion. It is now widely accepted that the cell responsible for histamine-mediated parietal cell activation is the ECL cell (6). These enteroendocrine cells are intimately associated with the much larger parietal cells and possess numerous vacuoles containing histamine, which is released by exocytosis. Cell surface receptors for gastrin and acetylcholine have been purified from ECL membrane preparations, suggesting that the ECL cell directly integrates these inputs and releases histamine in a paracrine fashion (Fig. 72-3).
The major inhibitory regulator of ECL cell function is somatostatin, which causes receptor-mediated suppression of histamine release in response to the secretagogues mentioned earlier. Somatostatin-secreting D cells also exert a tonic inhibitory effect on acid secretion by directly suppressing parietal cell function. The principal stimulators of D-cell activity are hydrogen ion, resulting in a negative feedback loop, and noncholinergic neurons, releasing VIP in response to low-grade distention. Other stimulators of fundic somatostatin release include gastrin and epinephrine, but their roles have not been clarified. Acetylcholine exerts a negative effect on somatostatin secretion, potentiating cholinergic enhancement of acid secretion by ECL and parietal cell stimulation.
The dominant pathway of parietal cell activation is paracrine stimulation of parietal cells by ECL cell-secreted histamine. The more proximal mechanisms that transduce physiologic stimuli into ECL cell histamine release involve the production of gastrin by antral G cells and the transmission of impulses through gastric effector neurons of the enteric autonomic system directly to the ECL cells. G cells are responsive to two stimulatory neurocrine agents: acetylcholine from cholinergic neurons, and GRP from noncholinergic neurons. Vagal cholinergic activity governs gastrin release from both cephalic-phase (CNS) stimulation and high-level gastric distention (i.e., after ingesting a meal). GRP appears to mediate gastric-phase activation of G cells by luminal protein fragments, particularly aromatic amino acids (7). On stimulation by either acetylcholine or GRP, antral G cells secrete gastrin into adjacent capillaries. This neurocrine stimulation accounts for the entire gastrin response to central and local factors.
Gastrin is actually a heterogeneous collection of at least three peptides: big gastrin (34 amino acids), little gastrin (17), and minigastrin (14). Big gastrin predominates physiologically because it has the longest half-life. Actions of gastrin include the direct stimulation of ECL cells and potentiation of the parietal cell response to histamine. Proposed physiologic effects of gastrin are trophic effects on oxyntic gland mucosa, secretion of IF and pepsin, and augmentation of lower esophageal sphincter (LES) pressure, intestinal motility, and pancreatic exocrine secretion.
G cells are under constant, ambient inhibitory influences exerted by somatostatin secreted from adjacent D cells. Histologically, D cells are linked to G cells through cell membrane processes that abut directly on the G-cell plasma membrane. Somatostatin, released into the interface, binds to receptors on the G cells that downregulate gastrin secretion. Somatostatin secretion is stimulated by gastrin, resulting in a negative feedback loop. In addition to gastrin, somatostatin secretion is elicited by VIP released from noncholinergic neurons in response to lowlevel gastric distention (i.e., after partial emptying of a meal) (8). This constitutive inhibition by somatostatin must be blocked for maximal stimulation of gastrin secretion to occur. Acetylcholine inhibits somatostatin secretion, potentiating its direct stimulation of gastrin secretion.
Mechanism of Acid Secretion
Activating agents bind with high affinity to parietal membrane receptors that are linked to second messenger systems, resulting in either the generation of cyclic adenosine monophosphate (cAMP) or an increase in the intracellular calcium concentration ([Ca]i). The H2 receptor appears to be a typical seven-membrane segment, G-protein–coupled receptor that activates adenylate cyclase on the cytoplasmic surface of the plasma membrane, resulting in the hydrolysis of adenosine triphosphate (ATP) and generation of cAMP. cAMP then triggers the phosphorylation and activation of various cytoplasmic protein kinases (9). The critical component in this linkage is the stimulatory guanosine triphosphate-binding protein (Gs) that translates external receptor occupation to internal adenylate cyclase activation. Both acetylcholine and gastrin receptors result in increased [Ca]i, presumably owing to phospholipase C-dependent inositol turnover and protein kinase C activation, increased surface membrane Ca2+ conductance, or both. Receptors for inhibitory substances (somatostatin, PGE, opioids, EGF) are linked to an inhibitory G protein (Gi) that suppresses adenylate cyclase activity.
When the appropriate stimulatory signals converge on the parietal cell basolateral membrane, a number of distinct morphologic changes occur that coincide with acid secretion from the apical end of the cell. Cytoplasmic tubulovesicles, which have been shown to contain the quiescent proton pump, coalesce into a larger membrane system termed the secretory canaliculus, which communicates with the gastric gland lumen at the apical cell surface. At the same time, the K+-Cl– channel, which provides exchangeable K+ ions to the extracellular portion of the proton pump, is also inserted into the membrane of the secretory canaliculus. These changes occur within minutes of activation and persist throughout the entire period of increased acid secretion.
Although the sequence of events after the activation of cAMP is undefined, these events eventually result in the activation of the proton pump—an H+-K+-ATPase that effects an electroneutral exchange of H+ for K+ at the luminal surface of the secretory canaliculus. Omeprazole, a substituted benzimidizole, is a weak base that becomes protonated and trapped within the low-pH canaliculus. There, it undergoes conversion to a sulfenamide, which reacts with the exposed portions of the catalytic subunit of the H+-K+-ATPase pump, forming stable disulfide bonds and preventing the conformational change that allows for ion transport.
Other Gastric Secretory Products
Pepsinogen
Pepsin refers to a family of digestive enzymes synthesized and secreted by the chief and mucous neck cells of the gastric glands. The pepsinogen family can be divided into two groups: A (or I), and C (or II). Pepsinogen A has a molecular weight of about 43 kd and is secreted by the proximal stomach; pepsinogen C is of similar size and is secreted by the entire gastric mucosal surface as well as by the duodenum, prostate, and seminal vesicles. The enzymes are actually synthesized and stored in zymogen granules as prepepsinogens, which undergo intracellular conversion to their zymogen forms, pepsinogens, by cleavage of an N-terminal sequence before secretion through exocytosis. In addition to pepsinogen A and C, two other acid proteinases are secreted by the gastric mucosa: cathepsin D and E. Their physiologic importance is not well defined.
After secretion as an inactive zymogen, an N-terminal sequence is cleaved by acid-mediated hydrolysis, converting pepsinogen to active pepsin. Thereafter, pepsin autocatalyzes its own conversion from pepsinogen. Pepsin is an acid proteinase that has an abundance of aspartate residues at the catalytic site (hence, an aspartic proteinase). At pH below 5, pepsin is progressively more efficient at cleaving internal peptide bonds between aromatic amino acids, thus generating protein fragments with exposed aromatic residues known to stimulate gastrin secretion maximally by GRP-dependent neurocrine stimulation. Pepsinogens can be detected in the fetal stomach by 8 weeks’ gestation, but peptic activity is absent until about 16 weeks.
Stimulation of pepsinogen secretion is primarily governed by cholinergic and β-adrenergic inputs. Paracrine and endocrine stimulation also occurs through histamine, CCK, secretin, and VIP. Gastrin does not elicit pepsinogen secretion. Inhibitory regulation is mediated by somatostatin and PGE.
Lipase
Gastric lipase activity is demonstrable at birth. Its optimal pH for activity (5.5) is higher than that of pepsin, but is still slightly acidic. Intragastric lipolysis liberates long-chain fatty acids, which stimulate duodenal CCK secretion, and medium-chain fatty acids, which are directly absorbed through the gastric epithelium.
Intrinsic Factor
Intrinsic factor is a 60-kd mucoprotein that is secreted from parietal cells in the fundus and body. Regulatory controls for IF closely parallel those for acid secretion, suggesting a physiologic coupling of IF and acid secretion. In the infant and young child, however, this coupling is dissociated, allowing a constant, basal secretion of IF, which exceeds that of acid (10).
In the stomach, IF competes with a second ligand, R protein, for binding to vitamin B12 cobalamins. Although vitamin B12 preferentially binds R protein in the stomach, pancreatic enzymes in the intestine degrade R protein, allowing IF to bind available vitamin B12. The IF-B12 complex is then absorbed in the terminal ileum.
Mucus
Mucus is a complex gel consisting of mucin, desquamated epithelial cells, salts, and water. In the stomach, bicarbonate is also secreted and trapped in this gel and is an important protective component of gastric mucus. Mucin, consisting of proteins, glycoproteins, and mucopolysaccharides, is secreted by surface epithelial cells and mucous neck cells in a manner that appears coupled to acid secretion—synthesis and secretion are stimulated by acetylcholine, histamine, and gastrin (11). The mucus layer is about 180-μm deep and displays a thin layer of hydrophobic phospholipids on its luminal surface.
The physiologic function and importance of mucus is controversial. Previously, the mucus–bicarbonate barrier was considered important in maintaining a pH-neutral environment overlying the surface epithelium by preventing back-diffusion of HCl and pepsin, and by preventing the dissipation of epithelial-secreted bicarbonate. Indeed, a pH gradient of nearly 5 logs exists between the gastric lumen and the epithelial surface. Disruption of the mucus layer by mucolytic agents, however, does not render the underlying epithelium more susceptible to acid-mediated injury, but it does exacerbate epithelial disruption by other injurious agents. It may be reasonable to conclude that the physiologic role of gastric mucus is not in preventing injury, but in protecting the already injured epithelial surface during the regenerative phase. Other functions of mucus include lubricating the gastric surface to minimize mechanical trauma during mixing, and trapping ingested microbes until they can be processed and phagocytized by immunocompetent cells.
Mucosal Defense Mechanisms
Mucosal defense is a critical ongoing function for an organ whose luminal surface is regularly exposed to a highly acidic environment, a wide range of osmolarities, and activated proteolytic enzymes. The components of mucosal defense, their relative importance, and the precise mechanisms by which they confer protection to the gastric epithelium are, however, poorly understood. Certain mechanisms have been proposed to constitute a hierarchical system of mucosal defense. Those that are best described include (1) mucus and bicarbonate secretion, (2) epithelial resistance and restitution, (3) mucosal blood flow, and (4) subepithelial activity of immunoinflammatory cells.
CONGENITAL ABNORMALITIES OF THE STOMACH AND DUODENUM
Abnormal Gastric Fixation and Gastric Volvulus
Congenital deficiencies of mesenteric fixation of the stomach to the surrounding structures predispose to gastric volvulus and can take two distinct forms. Normally, the stomach is suspended in a peritoneal leaflet that is tethered in four quadrants by the gastrohepatic, gastrophrenic, and gastrosplenic ligaments and the retroperitoneal fixation of the duodenum. The gastrocolic ligament (greater omentum) may also provide stabilization. Absence or laxity of the gastrohepatic and gastrosplenic ligaments allows the stomach to rotate around its longitudinal axis, producing an organoaxial volvulus (Fig. 72-4). Similar abnormalities of the gastrophrenic ligament and duodenal attachments allow rotation around the stomach’s transverse axis, referred to as a mesentericoaxial volvulus. Organoaxial volvulus is the more common of the two types in infants and children.
A strong association has been found between gastric volvulus and several other developmental anomalies. These include malrotation, asplenia, and congenital abnormalities of the diaphragm (e.g., hiatal hernia, posterolateral “Bochdalek” hernia, eventration) (12). Because these entities all result in abnormalities of the stabilizing peritoneal attachments listed earlier, a causative role is assumed. Gastric volvulus may also be associated with conditions resulting in the stretching and laxity of the peritoneal attachments caused by gastric distension (e.g., aerophagia, prior hypertrophic pyloric stenosis).
Although gastric volvulus can occur as either an acute or chronic problem, the acute form is more common in children. The classic presentation of sudden catastrophic epigastric pain, intractable retching without emesis, and inability to advance a nasogastric tube into the stomach is rarely encountered in the actual clinical setting. Children may experience emesis, which can be bilious or nonbilious, and may not have abdominal distention. Intermittent gastric volvulus may be considered in the workup of infants presenting with apparent life-threatening episodes (13). Any combination of symptoms suggesting a partial or complete proximal mechanical obstruction may be present. Profound physiologic decompensation, hemodynamic instability, or unrelenting metabolic acidosis suggest strangulation, ischemic necrosis, and possibly perforation. Obviously, such a presentation mandates emergent surgical intervention concurrent with ongoing resuscitation. Older children may present with chronic, intermittent volvulus exhibited by postprandial pain, early satiety, and belching.
Radiologic assessment can reveal several characteristic findings. On plain abdominal radiographs, massive gastric dilation can usually be seen, often with a distinct incisura pointing toward the right upper quadrant. The spleen and small intestine may be displaced inferiorly. If a contrast study has been attempted, the contrast column may be confined to the esophagus, with a long, gradual tapering at the bottom. Occasionally, a paraesophageal hiatal hernia is detected (Fig. 72-5).
Chronic idiopathic volvulus may be medically managed in selected cases (14). Operative treatment of acute gastric volvulus includes gastric decompression by nasogastric suction or needle aspiration and reduction of the volvulus. Perforations are closed primarily or, depending on their location, around a Malecot tube; more extensive necrosis requires resection. Coexisting anomalies, such as malrotation and diaphragmatic defects, should be corrected. Recurrent volvulus is prevented by performing an anterior gastropexy. Historically, a Stamm-type gastrostomy has often been employed to increase the stability of the gastropexy, although there are no data that suggest this is mandatory. Both laparoscopic and open approaches are effective. Recurrence is rare.
Microgastria
Congenital microgastria is a rare anomaly in which the stomach retains a tubular morphology, fails to develop its normal rotation and fixation, and attains a much smaller volume than normal. Follow-up involving one long-term survivor has documented that the gastric volume remains small indefinitely (15). Other abnormalities of the abdomen frequently accompany this condition, including megaesophagus, gastroesophageal reflux (GER), intrinsic duodenal obstruction, malrotation, biliary anomalies, situs inversus, asplenia, and skeletal defects.
Microgastria presents with vomiting and failure to thrive in the infant, often associated with persistent diarrhea. Vomiting may be due to gastric insufficiency, GER, or duodenal obstruction. Diarrhea is presumed to be due to rapid gastric transit and dumping. The diagnosis is established by contrast upper GI tract study, which reveals a small stomach with a transverse lie and frequently a large, patulous esophagus (Fig. 72-6). Particular attention should be given to the anatomy of the duodenum and ligament of Treitz.
The initial management of microgastria is usually by continuous drip feeding. This approach has been variably successful in providing adequate fluids and calories. In most cases, parenteral nutrition is necessary. When continuous feedings can be maintained for several weeks, the stomach may undergo significant enlargement, allowing for a gradual transition to bolus and ad lib feeds. Antireflux precautions, including small frequent meals, may be required indefinitely. If this strategy is unsuccessful, fundoplication may be necessary, and may require a more limited procedure (e.g., Thal or Dor gastropexy). Although case reports of successful management by gastrojejunostomy exist, the favored approach involves construction of a Roux-en-Y jejunal reservoir—the Hunt-Lawrence pouch (16).
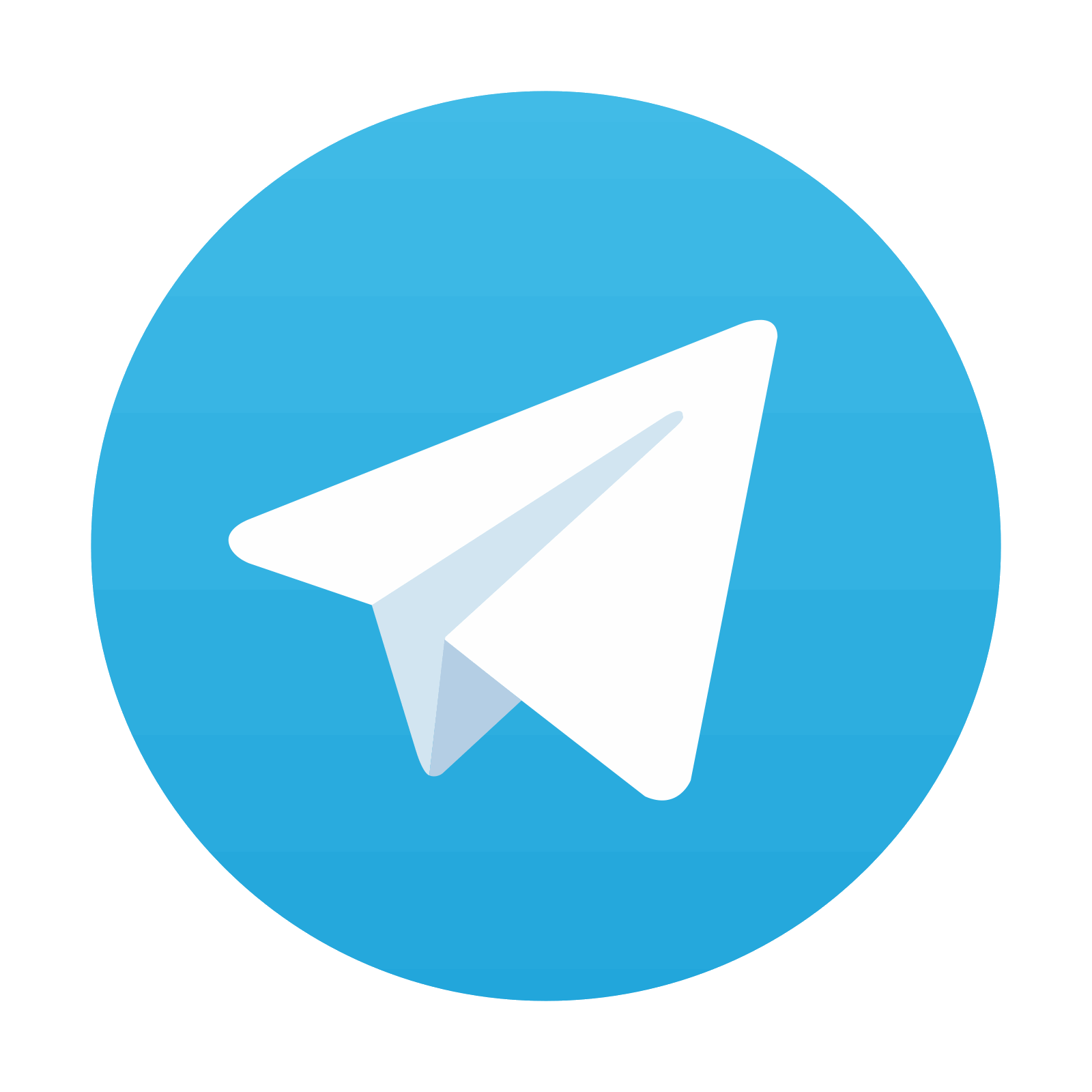
Stay updated, free articles. Join our Telegram channel
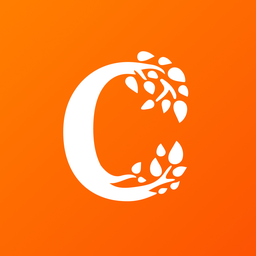
Full access? Get Clinical Tree
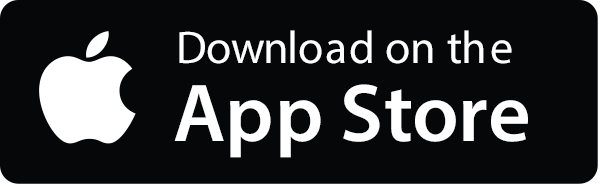
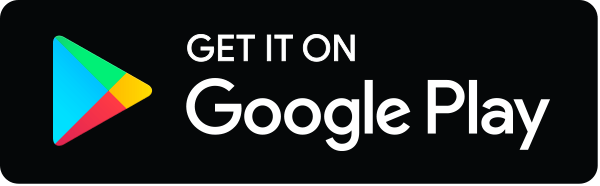