Radiologic Imaging of the Neonate
Jeffrey C. Hellinger
Nicole Mendelson
Mary M.K. Seshia
Mhairi G. MacDonald
Radiologic imaging is an essential clinical tool for investigating and monitoring neonatal disease. These diagnostic data are fundamental to prompt bedside management and decision making, particularly in a critically ill neonate in the intensive care unit (ICU). Goals are to obtain reliable and reproducible images that meet or exceed established standards for neonatal imaging. Images of sufficient quality should readily afford analysis, understanding, and accurate interpretation. While high diagnostic quality is a prerequisite for meaningful use and confident decision making, anatomical and functional neonatal imaging algorithms should always strive for the highest safety of the neonate.
Safety considerations in neonatal imaging include the type of imaging modality, the location of imaging, and the effectiveness of communication between all providers in the care of the neonate. Plain film radiography, ultrasound, fluoroscopy, computed tomography (CT), and magnetic resonance imaging (MRI) are common modalities utilized for neonatal imaging. Nuclear scintigraphy is less commonly used. Each modality has intrinsic benefits, risks, and limitations, which must be considered when choosing an imaging strategy and applying the interpretive findings. A primary risk consideration in neonatal imaging is radiation exposure (1). Radiography, fluoroscopy, CT, and nuclear scintigraphy are dependent upon radiation to generate images, while ultrasound and MRI are not. Another risk consideration is the transportation for and performance of imaging outside of the neonatal unit. Radiography and ultrasound can be performed routinely at the bedside, minimizing risks extrinsic to the type of modality. Fluoroscopy, MRI, and nuclear scintigraphy require transportation of the neonate to a dedicated radiology department, increasing the potential risks. While in select centers it is possible to perform CT at the bedside with a portable CT unit, in most neonatal centers CT is also performed in a dedicated imaging department.
Effective communication among bedside clinicians, radiologists, imaging technologists, and other health care providers is important to ensure appropriate use and performance of these modalities in neonatal imaging. When modalities are applied and operated appropriately, the potential for diagnostic yield, clinical impact, and neonatal safety is optimized. Effective communication is achieved through a collaborative team approach to share a neonate’s clinical data. In this manner, imaging protocols and strategies are best tailored to the neonate’s clinical presentation and known or suspected disease process, while the duration and volume of imaging are potentially minimized. It is equally important that there is clear and timely reporting of findings to optimize timely and appropriate bedside decision making. Full-proof protocols for conveying urgent imaging diagnoses from the radiologist to the NICU team must be in place and, in reverse, ready access to a radiologist for consultation. In the current digital era, it is strongly recommended that the neonatal imaging team, with all relevant health care providers, reserve direct personal time for regular consultative discussions and reviews of patients, their pathologic processes, clinical management, radiologic studies, and other diagnostic data. Strong emphasis on communication and teamwork is also paramount in order to minimize risks to the neonate during transportation for radiologic studies outside the neonatal unit.
Imaging of the neonate is distinguished by the small size of the patient, immature nature of organs, distinct physiology, range of potential multiorgan congenital disorders, and unique in utero and early postpartum acquired pathology. These unique characteristics present technologic and interpretive challenges for the imaging team as it seeks optimization of performance, patient safety, and diagnostic yield, along with minimization of cost and potential short- and long-term iatrogenic sequelae from imaging. These challenges further highlight the importance of strong teamwork, communication, and consideration of the risks and benefits of the radiologic modalities. Addressing these challenges and successfully utilizing multimodality diagnostic imaging for neonatal clinical management require fundamental understanding of neonatal imaging principles, the imaging modalities, and key modality findings for neonatal pathology. This chapter provides a review of imaging principles and modalities and an overview of imaging findings for neonatal pathology using a body system approach. Also included is a review of the radiologic evaluation of neonatal catheters and other support devices.
Neonatal organs are highly sensitive to radiation, relative to more mature organs in older children and adolescents and the fully developed organs in adults. Organs, however, have variable radiosensitivity. Targeted imaging with the radiation-dependent modalities results in an additional source of variable organ exposure. Despite neonatal organs having variable exposure and susceptibility, one must remember that radiation exposure is cumulative (2). Each time a neonate undergoes an examination with a radiation-dependent modality, the output effective radiation dose is added to the total effective exposure. The neonate’s total effective radiation dose is then measured against natural background exposure that the neonate may experience from the environment. The natural radiation exposure averages approximately 2.5 millisieverts (mSv) per year (1).
A fundamental principle for neonatal imaging is to restrict radiation exposure to not more than 2.5 mSv per year. This may not be possible for all neonates as a neonate’s disease process and clinical management may dictate frequent imaging and higher utilization of radiation-dependent modalities (2). Nevertheless, every attempt should be made to control a neonate’s exposure to radiation from medical imaging and reduce the potential adverse end-organ sequela.
Restricting a neonate’s medical radiation exposure requires that neonatologists and radiologists adhere to the principles of ALARA— As Low As Reasonably Achievable (3). Foremost to achieving ALARA in one’s clinical practice is to selectively limit the use and volume of studies dependent upon radiation. Based upon the clinical need, this mandates primary consideration of first employing a nonradiation imaging modality (e.g., ultrasound or MRI) or a nonimaging solution for diagnosis. If there is no alternative to a radiation-dependent modality, performance should follow established guidelines in an effort to keep exposure low (3,4). Core tenets to these guidelines are that imaging should always be based upon sound clinical indications, a high pretest diagnostic yield probability, and moderate-to-high potential clinical impact from the imaging findings. Repeat (e.g., follow-up head CT) and routine examinations (e.g., daily chest radiographs) should be limited and performed only when the benefits of radiation exposure will outweigh the risks of added effective radiation exposure. When imaging is performed with a radiation modality, core technical strategies are employed to reduce neonatal radiation exposure. These include (a) weight-based protocols for appropriate radiation imaging parameters (e.g., kilovoltage, kV; milliamperage, mAs) and (b) body and selective organ-protective shielding (3,4).
While it is always possible to image at the lowest possible kV and mAs parameters, the penalty may be nondiagnostic quality images. Nondiagnostic images can also result from incorrect neonatal positioning, neonatal motion, and overlying support devices (Fig. 6.1). The result in these instances is a nondiagnostic exam and ineffective utilization of health care resources and time. More importantly for neonatal safety, these nondiagnostic exams also result in “unnecessary” radiation exposure, which has no clinical value and only adds to the neonate’s cumulative effective dose and risk. In other instances, the radiation settings may render an exam of diagnostic quality for the radiologist to interpret, but of insufficient quality for the bedside clinician to confidently make decisions. The result is also an ineffective use of resources and an unnecessary exposure to radiation; the exposure does not lead to decision making and potential changes in the neonate’s medical management. At the other end of the spectrum, complete avoidance of radiation-dependent modalities and reliance solely upon ultrasound and MRI are not cost-effective, nor workflow efficient. For some disease processes, a radiation-dependent modality will have a higher diagnostic performance. Not utilizing a radiation modality because of concern about radiation exposure could then also adversely impact resource utilization and clinical management.
Quality control measures are critically important to balancing the appropriate use of radiation-dependent modalities and adhering to ALARA. Radiology departments in collaboration with the neonatologists should have pathology-based protocols, outlining recommended modality workflow algorithms. This is based upon scientific performance and the modalities’ risks, benefits, and alternatives. Radiologists should have recommended positioning and radiation exposure parameters (e.g., kV, mAs) for technologists to apply when employing a radiation-dependent modality. Instructions for general body and selective organ shielding (based upon the modality and region scanned) are additional radiation control measures. Finally, when preparing for the exam, control efforts should also focus on reducing or eliminating potential sources of poor image quality. Support devices nonessential to the imaging goals should be excluded from the field of view if possible. Neonatal motion can be controlled by swaddling or use of sucrose in some examinations (5). For other examinations, sedation is key to controlling motion. Sedation protocols should be established in collaboration with neonatologists and pediatric anesthesiologists for optimum results and patient safety. Third-party products and software are available to record and monitor a neonate’s cumulative exposure. Knowledge of a neonate’s actual cumulative dose can help restrict medical radiation exposure and guide imaging strategies.
Continuous quality reviews are fundamental to ensuring appropriate modality utilization and compliance with control measures. They are also important to ensuring that exams are of diagnostic quality and are of sufficient quality for rendering both interpretations and confident clinical decisions. Reviews should assess all aspects that may impact exam quality including but not limited to radiation exposure settings, positioning, support devices, and motion. Three hundred sixty-degree input and feedback from the entire extended imaging team are essential to assessing the quality of the neonatal imaging program, identifying areas for improvement, and implementing changes that will provide optimal neonatal clinical care and safety.
A final set of general principles for neonatal imaging regards interpretation of the study. For the radiologist and neonatologist, a systematic analytical approach to each type of imaging study will ensure complete assessment of neonatal anatomy and identification of potential abnormal pathology and pathophysiology. Evaluation begins with image quality, proceeds to the support devices in the imaged field of view, and then finally the imaged anatomy. Recognition of factors that may degrade image quality is a primary step as these factors will potentially negatively impact the exam’s sensitivity, specificity, and positive and negative predictive values. Knowing the limitations of the exam helps establish a level of confidence in detecting support devices, depicting and identifying abnormal anatomy, and making subsequent management decisions. Determinants of image quality will vary by modality. Support devices are recommended as the second step so that these catheters, tubes, and wires are not overlooked in the diagnostic analysis. Recognition of a malpositioned device and potential iatrogenic neonatal complication can only be made by actively searching. Support devices may also provide insight to a neonate’s congenital disorder, postpartum acquired disease, clinical management, or a combination thereof. In the third step, neonatal anatomy is evaluated. Core review addresses size, shape, and contour of the imaged anatomy. More specific anatomical and functional reviews and findings will vary by the modality and disease process.
Radiography
Radiography is a two-dimensional (2D) static x-ray imaging technique that depicts structures based upon relative densities (e.g., air, fat, water, soft tissue, bone). Radiodense structures (e.g., bone), devices, and foreign bodies are well depicted. Visceral silhouettes and soft tissue lines are depicted with limited detail based upon the adjacent differential densities.
Radiography is the most commonly used modality in neonatal imaging and has historically been and remains one of the first steps in radiologic investigations for most neonatal disease processes. The advantages of radiography are that it is a portable exam, which is easy to perform, process, and interpret in a rapid manner. This makes it a highly effective method to rapidly screen for neonatal pathology and monitor medical and postprocedural treatment responses involving the neck, chest, abdomen-pelvis, and skeletal structures. The evaluation of support device positioning is a further key indication for obtaining radiographs in the neonate. In each of these applications, anatomy outside of the region(s) of interest should always be shielded.
For some clinical presentations, single-anatomical-level radiography is appropriate. For example, a noncyanotic neonate with intermittent tachypnea will initially undergo an anteroposterior (AP) chest radiograph. A neonate with abdominal distention and vomiting but no respiratory distress will initially undergo an AP abdominal-pelvis radiograph. A hemodynamically stable neonate with a suspected osseous congenital anomaly will undergo targeted bone imaging (e.g., forearm for radial array anomaly). For more critical neonates with greater time-sensitive diagnostic needs, multilevel radiography is appropriate. For example, for a neonate in respiratory distress, a combined AP chest and abdominal-pelvis radiograph is performed. This facilitates evaluation of targeted anatomy with categorization of visceral situs, recognition of potential cardiovascular and noncardiovascular congenital disease, and confirmation of appropriate positioning of all support devices placed during initial neonatal resuscitation and evaluation. Subsequent radiographs can then be targeted to a single level of concern, for example, chest or abdomen-pelvis radiograph. Follow-up chest radiographs should extend to at least the upper abdomen to account for variable degrees of inspiration and confirm stability of support devices. For example, in Figure 6.1, had the upper abdomen not been included in this well-centered chest x-ray, the tip of the doubled back nasogastric tube might have been mistaken for the tip of an umbilical arterial catheter (UAC). The acute clinician would recognize, however, that the UAC if properly placed runs parallel with the left side of the spine on an AP film and would not deviate to the right as shown. When support devices require complete follow-up evaluation, coverage should again extend across multiple anatomical levels to include their entire anticipated locations. At times, lateral projections may be obtained in conjunction with the standard AP radiographs to evaluate anatomy, suspected pathology, and/or confirm positioning of support devices (Figs. 6.2 and 6.3). Diagnostic quality of radiographs may be degraded by incorrect positioning, motion, overlying devices, and the degree of under- or overexposure (Fig. 6.4B). In addition, low inspiratory volumes may degrade the evaluation of chest radiographs.
Appropriately used radiography exposes the neonate to the least amount of radiation, as compared to other radiation-dependent modalities. The typical dose from an AP chest radiograph is 0.02 mSv, while the dose from a combined AP chest-abdominal-pelvis radiograph is up to 0.12 mSv (2). These values can be applied as a means to understand a neonate’s dose exposure from other radiation modalities and also when assessing a neonate’s cumulative dose. In this manner, dose exposure is expressed as an equivalent number of radiographs (e.g., chest radiographs). While this approach may provide a measure for communicating and understanding a neonate’s exposure and risk for single and cumulative exams, it is not always an accurate means for reporting exposure. As previously discussed, organs may have differential exposure, depending upon coverage, organ radiosensitivity, and shielding.
Ultrasound
Ultrasound is a modality that utilizes high-frequency sound waves to generate anatomical images and functional data. B-mode “gray-scale” real-time sonography serves as a primary method to investigate neonatal congenital and acquired pathology across most anatomical regions. This includes assessment of intracranial, intra-abdominal-pelvis, cardiovascular, and musculoskeletal pathologies (Fig. 6.5). Additional use of gray-scale ultrasound includes image guidance for cardiovascular and noncardiovascular interventions (Fig. 6.6). Color and pulse-wave Doppler sonography are supplemental techniques that afford evaluation of cardiovascular physiology. Doppler sonography is also used to evaluate ureteral flow and exclude uterovesicular obstruction. M-mode sonography can be applied along with gray-scale sonography to evaluate diaphragmatic excursion and quantify the degree of motion. Three-dimensional (3D) ultrasound techniques provide volumetric data and sophisticated morphologic displays, complementary to standard 2D displays.
The key advantage of ultrasound is that it is does not require ionizing radiation to generate images and physiologic data. In addition, it is widely available, is portable, and can be performed rapidly at the bedside in the neonatal ICU. The sonographic window may be degraded by catheters, metallic devices, bone, anatomical deformities, soft tissue edema, body fluid, and normal and abnormal regions of air. Sonography may also be limited by operator skill, emphasizing the importance of continuous quality control and review.
Fluoroscopy
Fluoroscopy is a rapid sequential 2D x-ray imaging technique that affords dynamic real-time imaging. As with static radiography, fluoroscopy depicts structures based upon the relative densities of air, fat, water, soft tissue, and bone. Radiodense structures (e.g., bone), devices, and foreign bodies are well identified, while viscera and soft tissues are depicted with limited structural detail. Oral, intracavity, and direct intravascular iodinated water-soluble contrast are administered to enhance lumens, generate new density interfaces, and increase structural depiction and detail. Multiple specific technical radiation reduction strategies are used simultaneously during fluoroscopy, in conjunction with the previously described core strategies. These include (a) using intermittent, pulsed x-ray beams (as opposed to constant and continuous fluoroscopy) of narrow width and low rates; (b) capturing the majority of the exam with “last image hold” and “fluoroscopic save” options (as opposed to actual radiographic images); (c) using x-ray beam and field-of-view filters; and (d) selecting appropriate larger field of views and greater radiation source to skin distances. (3) In addition, antiscatter grids should be used on a limited basis.
Common neonatal applications of fluoroscopy include evaluations of the gastrointestinal (GI) tract and genitourinary system. Typically, these exams follow an ultrasound of the abdomen, pelvis, or both. Fluoroscopic evaluation of the GI tract requires intraluminal contrast opacification. Once opacified, GI tract shape, luminal integrity, course, caliber, and contour can be assessed. For the upper and mid-GI tract, oral contrast is administered (e.g., barium swallow, esophagram, upper GI series, or small bowel follow-through); the transit of contrast is monitored in an antegrade direction. For the lower GI tract, a contrast enema is administered in retrograde fashion toward the cecum (e.g., barium enema). Performance of the appropriate fluoroscopic GI exam and selection of the correct type and volume of contrast will depend upon the neonate’s clinical presentation and suspected pathology. Neonatal dose exposures from an upper GI series and barium enema may be as low as 0.5 and 0.4 mSv, respectively (2).
The neonatal genitourinary system is evaluated under fluoroscopy, most commonly with voiding cystourethrography (VCUG). For this examination, contrast is instilled into the bladder in a retrograde fashion via a catheter. Images are acquired during bladder filling, maximal distention, and voiding to assess bladder and urethra morphology and exclude ureteral reflux, urethral obstruction, and congenital anomalies involving the bladder, ureters, and urethra (Fig. 6.7). VCUG catheterization should always be performed by a skilled provider using proper, sterile technique. Neonatal dose exposure from a VCUG may be as low as 0.1 mSv (2).
Historically, fluoroscopy has also been used to evaluate diaphragmatic motion, airway morphology, and the cardiovascular system. Fluoroscopy combined with endoscopy has also been used for diagnostic evaluation of neonatal biliary and pancreatic ducts (e.g., endoscopic retrograde cholangiopancreatography [ERPC]). Fluoroscopy is still an option to evaluate a neonate’s diaphragm and airway. However, ultrasound and MRI are now more common, nonradiation alternatives to evaluate the diaphragm, while both MRI and CT can be used to evaluate the neonatal airway. In most neonatal centers, MR cholangiopancreatography has replaced ERCP, while ultrasound, MRI, magnetic resonance angiography (MRA), and CT angiography (CTA) are now principle noninvasive cardiovascular imaging modalities. Fluoroscopic-based invasive catheter angiography is performed selectively in neonates to investigate congenital heart disease (CHD), congenital vascular disorders (CVD), and acquired cardiovascular pathologies (see Chapter 30, Figs. 30.22, 30.24, and 30.26).
Fluoroscopy remains a primary technique for image-guided cardiovascular and noncardiovascular interventions in the neonate. Fluoroscopic guidance may be combined with ultrasound to improve technical guidance and potentially decrease radiation exposure. Hybrid CT-fluoroscopy and MRI-fluoroscopy technologies are also available and can be applied for neonatal image-guided interventions. Cardiovascular applications include vascular access and interventions for CHD, CVD, and acquired pathologies. Potential noncardiovascular applications include enteric access (e.g., feeding and percutaneous gastrostomy tube placements), percutaneous transhepatic cholangiography and biliary drainage, percutaneous cholecystostomy, and percutaneous nephrostomy. Noncardiovascular applications also include intraoperative guidance in select surgical procedures. Radiation exposure during procedures will depend upon the complexity of the interventional procedure, extent of disease, comorbid risk factors, and the operator’s technique.
Computed Tomography
CT is a multiprojectional x-ray beam technique that generates 2D cross-sectional images in the z-axis direction across all body systems. With current CT scanners, submillimeter sections can be acquired. These datasets afford multiprojectional 3D anatomical reconstructed displays that enhance interpretation, anatomical understanding, and treatment planning. High spatial resolution and superior tissue characterization, combined with frequent exam availability, rapid scan times, and ease of patient access make CT a desirable modality in select neonatal pathologies. CT scan times in a neonate may range from less than 1 second to 5-8 seconds depending upon the CT scanner technology, range of coverage, and prescribed technique. While motion can degrade CT image quality, more rapid CT acquisitions may afford imaging of the neonate independent of motion and without the need for sedation.
The radiation risks posed by CT are the greatest deterrents to its use in neonates. However, submillisievert exposure is possible when utilizing core and advanced CT-specific radiation reduction strategies (4). CT often follows ultrasound or fluoroscopic diagnostic evaluations. Based upon its advantages, CT imaging is indicated in the neonate for emergent evaluations (e.g., head CT for investigation of intracranial hemorrhage [ICH], ischemia, or trauma); for superior anatomical characterization (e.g., chest CT for congenital lung lesions) (see Chapter 41, Fig. 41.15); and for a neonate who has high sedation or general anesthesia risks. CT is used to define choanal atresia (see Chapter 41, Fig. 41.5A). CT is also indicated when MRI is not available, is contraindicated (e.g., during extracorporeal membrane oxygenation), is nondiagnostic (e.g., ferromagnetic materials—patent ductus arteriosus [PDA] clip), or has a high pretest probability of yielding a nondiagnostic exam. CT may be performed without or with iodinated intravenous contrast, depending upon the exam indications. Intravenous contrast-enhanced CT images may be acquired during the arterial phase, venous phase, or visceral phase, yielding CT arteriography, CT venography, or routine CT scans, respectively. An abdominal-pelvis CT may be performed in a neonate without or with oral water-soluble iodinated contrast.
Magnetic Resonance Imaging
MRI is an imaging technique that uses high magnetic fields and radio waves to generate 2D and 3D anatomical displays at any targeted plane for an imaged body system. Functional sequences can also be acquired to generate physiologic data. The lack of radiation and iodinated contrast exposure along with the ability to inherently delineate and characterize tissues with high contrast resolution should clearly make MRI an ideal choice for neonatal imaging.
In clinical neonatal practice, however, MRI is used as a secondary modality, typically following ultrasound or CT. In part, this is related to potentially long scan times and limited availability. More importantly, the majority of MRI exams in a neonate require conscious sedation or (less commonly) general anesthesia to control neonatal motion. This places added risks on the neonate. Not only can monitoring be challenging during the exam but the mechanical ventilation, oxygen, and anesthetics can profoundly alter the neonate’s circulatory physiology. Furthermore, anesthetics have been associated with potential neuroapoptotic effects in the brain of young patients (6) (see Chapter 53). In addition to motion, MRI quality may be degraded by metallic (e.g., ferromagnetic) structures and air. Preexam screening is essential to plan for sedation or general anesthesia, discuss the added risks with the family, and identify metallic objects and devices that may degrade image quality (see Chapter 46, Figs. 46.12, 46.14, 46.16, 46.17, 46.18, 46.19 and 46.20).
Nuclear Scintigraphy
Nuclear imaging is a technique that uses radioactive material (e.g., radiopharmaceutical) to map out cellular activity in 2D and 3D anatomical displays. The radiopharmaceutical is delivered to target a set of organs and organ system based upon cellular properties. Radiation is emitted from the organs to form an image, directly proportional to the organ uptake and metabolic activity. This affords an ability to evaluate normal and abnormal cellular physiology and indirectly, anatomic morphology. Primary considerations in the neonate include the diagnosis of biliary atresia (e.g., hepatobiliary scan) and the localization of a suspected intrapancreatic insulin producing tumor (e.g., positron emission tomography [PET]-CT exam). While evaluations of the brain, endocrine system, heart, lungs, kidneys (Fig. 40.16D), GI tract, and skeletal system are possible, other applications in the neonate are on a selective basis given the radiation exposure and ability of ultrasound, MRI, and low-dose CT to reliably provide a diagnosis. As an example of the potentially high radiation exposure with nuclear scintigraphy, a hepatobiliary scan in a neonate may result in exposure to 6 to 7 mSv (2). Because of high radiation exposure, nuclear scintigraphy is infrequently used for the neonate.
Catheters and Support Devices
Clinical care of a neonate in the ICU routinely leads to the placement of several different types of catheters, tubes, and other devices. Core neonatal ICU devices include endotracheal tubes (ETTs), UAC, venous access catheters, enteric access catheters, and intercostal catheters. Following placement of a device and before it is used, confirmation of suitable positioning is an immediate priority. Prompt recognition of an abnormally positioned device is critical for rapid management of a potential iatrogenic injury and prevention of short- and long-term sequelae.
While there are several clinical bedside measures which the clinician can employ to affirm appropriate positioning (e.g., auscultation and carbon dioxide monitoring for ETT placement), radiography serves an essential primary diagnostic role in device management. Device positioning can change during the clinical course of the neonate. Thus, periodic follow-up radiography serves a key secondary role in device management. Beyond these core clinical functions, radiographic assessment of support devices also serves an important ancillary role in the recognition and evaluation of congenital cardiovascular and noncardiovascular disease The abnormal course of several different devices can offer insight into presence of situs inversus, heterotaxy, esophageal atresia, congenital diaphragmatic hernia (CDH), abdominal wall defects, patent foramen ovale, an atrial septal defect, a persistent left superior vena cava (SVC), a persistent left inferior vena cava (IVC), a right aortic arch, and a circumflex descending aorta.
In addition to the general imaging principles previously discussed, there are a few radiographic principles specific for imaging devices. First, to ensure adequate depiction of the devices, kV and mAs radiation parameters may need to be adjusted from the typical neonatal settings. Second, the field of view should completely cover the internal as well as external segments of the device. This may require imaging multiple anatomical regions of the neonate (e.g., chest, abdomen, and pelvis). Third, all external segments should be isolated in a straight course, separate from other devices and aligned at an angle different from the expected internal course. For an AP radiograph, isolating external from internal segments may require manually repositioning the external segment or obliquely positioning the neonate. Fourth, all monitoring leads and other objects not related to the support device should be removed from the field of view. Fifth, when the course and final position of a device are indeterminate based upon a diagnostic-quality AP radiograph, an additional oblique or lateral projection must be obtained (Fig. 6.8A, and B). Alternatively, suboptimal image quality may preclude diagnostic assessment of the device. In this instance, the AP radiograph can be repeated with proper adjustments to correct for potential inappropriate radiation technique, positioning, overlying leads, or a combination thereof.
Endotracheal Tubes
Whether placed from an oral or nasal route, an ETT courses along the spine, ipsilateral to the neonate’s chin and is positioned ideally with the tip approximately 1 cm above the carina (7). The carina is readily identified on an AP chest radiograph and is used as a reference point to measure distances for required ETT advancement or retraction. When radiographically assessing the level of the ETT tip, consideration should be given to the neonate’s position. This is of particular importance in the extremely low-birth-weight infant. The ETT tip position will vary based upon neutral versus lateral positioning of the head and upward versus downward tilt of the chin. Lateral head and downward chin positioning each will result in the ETT tip located higher than neutral positioning, while an upward chin tilt will yield a lower ETT position. Aside from determining the ETT tip position, it is important radiographically to exclude bronchus intubation, esophageal intubation, and traumatic injury.
Bronchus intubation more commonly will occur into the right main bronchus, as it has a straight takeoff and course relative to the distal trachea (Fig. 6.9). The more horizontal alignment of the left main bronchus creates natural resistance should the ETT advance to the carina. With a right bronchus intubation, it is not uncommon for the ETT to extend further into the bronchus intermedius and potentially the right lower or middle lobes (Fig. 6.10). Critical ancillary findings on a chest radiograph may include left lung and right upper lobe atelectasis.
Clinically, esophageal intubation should be readily detected. With ventilation, there will be an absence of chest expansion, coinciding with a rise of the abdominal wall, a decrease in oxygen saturation, and an absence of exhaled carbon dioxide (8). AP chest radiographic depiction of esophageal intubation is facilitated by positioning the neonate’s head to the side. By offsetting the anterior trachea from the posterior esophagus, the ETT will be shown to course along the spine contralateral to the anterior chin.
During ETT insertion, mucosal tears or perforations to the pharynx or esophagus may occur. These traumatic injuries may not always be recognized at the bedside. Injury may be suggested radiographically when the ETT has an atypical course and location within the mediastinal silhouette. Perforation is diagnosed radiographically when there is pneumomediastinum or subcutaneous emphysema following ETT insertion. Another manifestation of airway injury is illustrated in Figure 6.11.
Vascular Catheters
Neonatal vascular access catheters that require radiologic imaging include UAC, umbilical venous catheters (UVC), and percutaneously inserted central venous catheters (PICC). UACs are critical to monitoring the neonate’s physiologic parameters and making management decisions. An UAC enters the umbilical artery and courses retrograde into an internal iliac artery and then into the ipsilateral common iliac artery to lie either in the supramesenteric thoracoabdominal aorta (T6-11) or in the infrarenal abdominal aorta (L3-4). Thoracic and lumbar spine landmarks are used radiographically to confirm appropriate positioning. UVC and PICC lines are placed for delivery of fluids, medications, and parenteral nutrition in addition to taking blood for laboratory analysis. UVCs are utilized in the early days following birth, while PICCs are used for more long-term venous access solutions. UVCs course from the umbilical vein to left portal vein and then cross into the IVC via the ductus venosus. The tip of the catheter ideally should be at the IVC-right atrial (RA) junction. This is readily identified radiographically. PICC lines are typically inserted in a peripheral upper extremity brachial, basilic, or less commonly a cephalic vein and course centrally to terminate at the SVC-RA junction. If the catheter cannot be advanced to the SVC-RA junction, the ipsilateral brachiocephalic vein is also acceptable. When radiographically assessing an upper extremity PICC line, it is important to keep in mind that the catheter tip position will vary depending upon arm abduction and adduction and the accessed vein. PICC lines may also be inserted via the common femoral vein. In this instance, the catheter courses centrally to terminate at the IVC-RA junction.
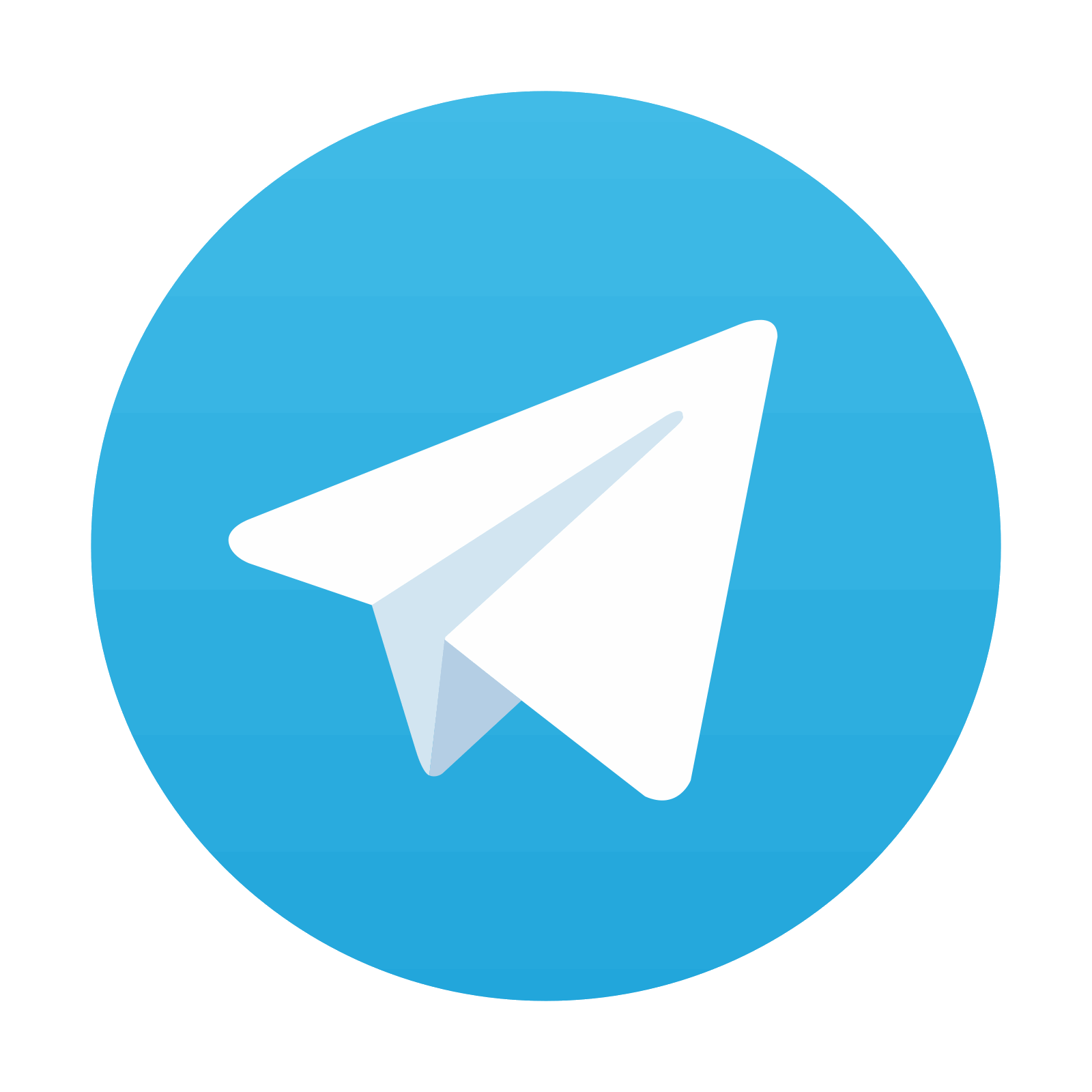
Stay updated, free articles. Join our Telegram channel
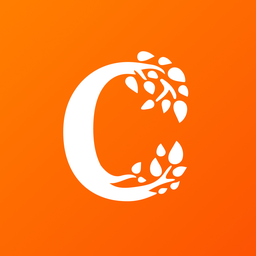
Full access? Get Clinical Tree
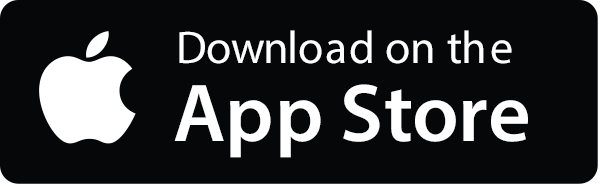
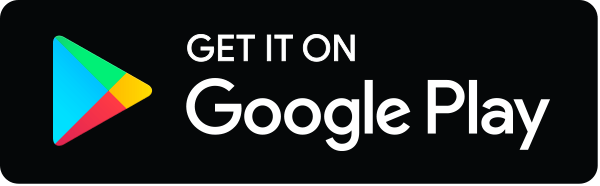