results do not support maintaining oxygen saturation levels in very preterm infants in the first weeks of life between 85% and 89%. This is still a very controversial area. Although lower saturations are not routinely recommended, high saturations may also have increased risks, so limiting high saturation alarm to 95% is a common recommendation for these preterm infants (1). Additional analyses are being conducted that will combine individual patient data from these studies in an effort to arrive at a more precise and timely recommendation for specific groups of patients based on gestational age, postnatal age, and perhaps other confounding factors.
able to increase the work of breathing to accomplish adequate gas exchange without treatment, including oxygen administration. The extremely premature infant will have a much weaker respiratory drive and inadequate muscular development and, thus, is less able to compensate for lung abnormalities. The classic clinical signs of respiratory distress are helpful in the assessment of the mature newborn infant. Nasal flaring, grunting respirations, and tachypnea are almost always present. With progression of lung disease and decreased lung compliance, chest wall retractions become more marked. With increased work of breathing, retractions progress from sternal to subcostal, to intercostal, and then to a seesaw pattern of chest and abdominal wall movement. The full-term infant may increase respiratory rate above 100 per minute, with shallow respirations. This pattern is the most efficient way to increase gas exchange, with the least costly work of breathing. Expiratory grunting represents an effort to retard expiratory flow to increase end-expiratory pressure and maintain alveolar patency. It is unsafe to rely on color changes as an indication of oxygenation, as abnormalities in peripheral perfusion as a result of poor cardiac output, hypotension, or hypovolemia may be misleading. Similarly, infants with recurrent apnea will have intermittent deficiency in gas exchange. Auscultation assists in determining the quality of air entry in various parts of the lung and the presence of airway secretions or obstructions.
spontaneous breathing or as positive end-expiratory pressure (PEEP) during mechanical ventilation. This usually requires pressures from at least 5 cm H2O to 8 cm H2O if the lung disease is severe.
TABLE 28.1 Risks of Hypocarbia and Hypercarbia | ||||
---|---|---|---|---|
|

tract results in a total airway resistance approximately 15 times greater than that of an adult (39). Edema and inflammation can produce extremely high resistance to air flow in these narrow airways. During expiration, the airways become narrower, and resistance increases.
of tube placement are intubation of the esophagus and intubation of the right mainstem bronchus. Auscultation, although helpful, is not reliable because breath sounds are well transmitted in a small chest. A chest radiograph should be obtained to confirm tube placement.
TABLE 28.2 Depth of Insertion of an Orotracheal Tube from the Lips of a Premature Infant | ||||||||||
---|---|---|---|---|---|---|---|---|---|---|
|

result in tidal volume distribution only to already overinflated lung regions, resulting in worsened (




inspiratory effort and provide the mechanical initiation of machineassisted ventilation during the early phase of the infant’s inspiration. Currently used methods to signal the initiation of inspiratory effort are listed in Table 28.4. The current methodology allows transduction to be accomplished in as short a time as 30 to 50 milliseconds, approximately one-tenth the duration of the inspiratory phase of a spontaneous respiratory cycle (Fig. 28.7) (50). The means by which this signal is provided and the addition of other subtle but potentially important changes in the capabilities of particular ventilators differentiate one type of conventional neonatal ventilator from another (51). A list of available modes and their theoretical advantages is found (Table 28.5).
TABLE 28.3 Management Considerations | ||
---|---|---|
|
TABLE 28.4 Available Mechanisms for Detecting Onset of Respiration | ||||||||||||||||||||||||||||
---|---|---|---|---|---|---|---|---|---|---|---|---|---|---|---|---|---|---|---|---|---|---|---|---|---|---|---|---|
|
machine-initiated tidal volume and flow patterns. Sensors monitor instantaneous flow rate and volume of gas from ventilator to patient; the applied pressure then changes according to the equation of motion. This system may allow for both greater patient comfort and reduction of peak airway pressure required to sustain ventilation, with less likelihood of overventilation compared to assist/control modes (52).
TABLE 28.5 Modes of Assisted Ventilation Via Endotracheal Tube | ||||||||||||||||||
---|---|---|---|---|---|---|---|---|---|---|---|---|---|---|---|---|---|---|
|
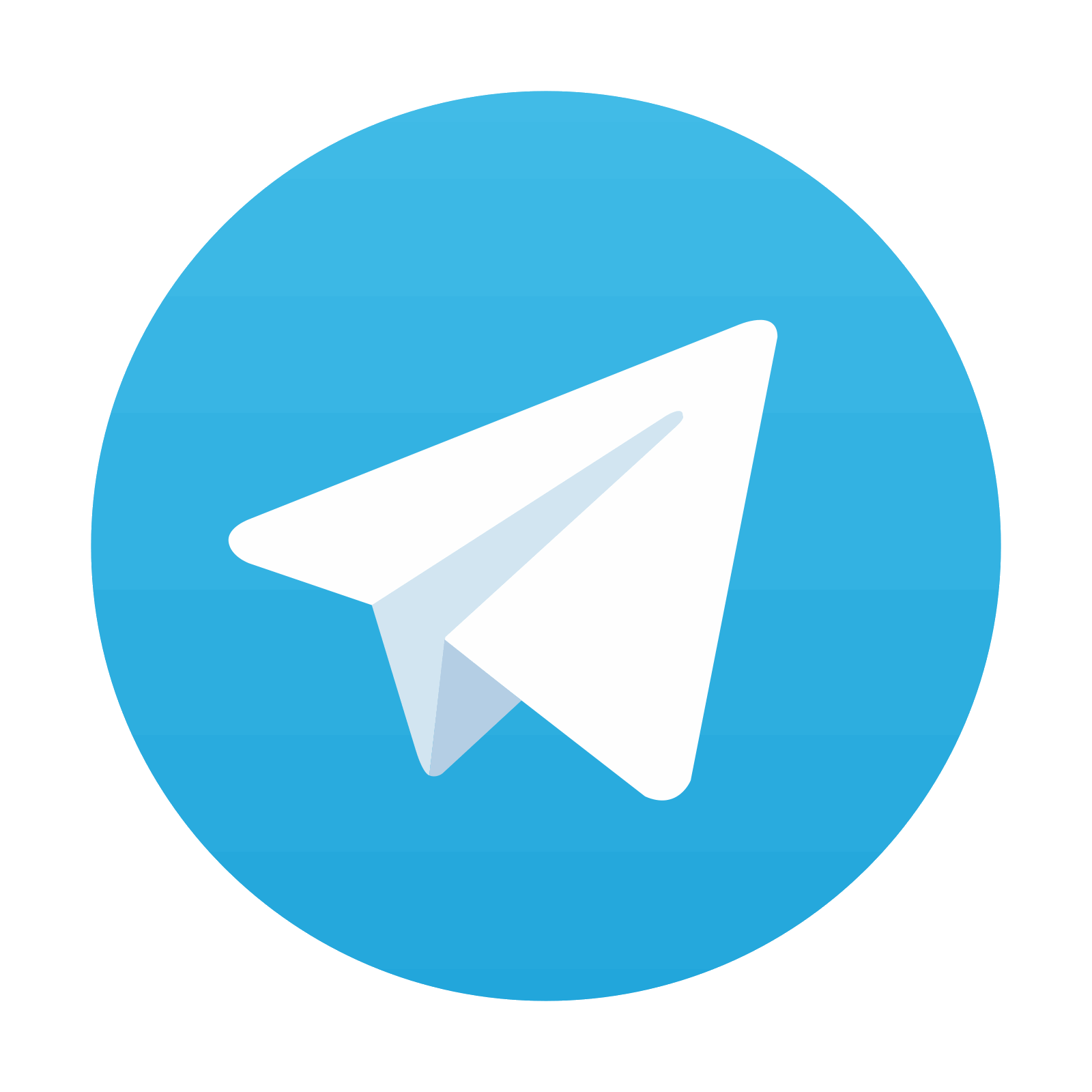
Stay updated, free articles. Join our Telegram channel
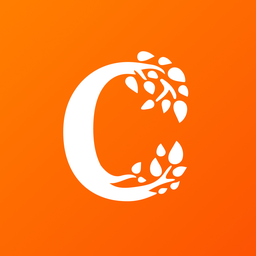
Full access? Get Clinical Tree
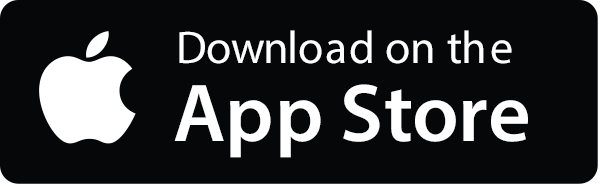
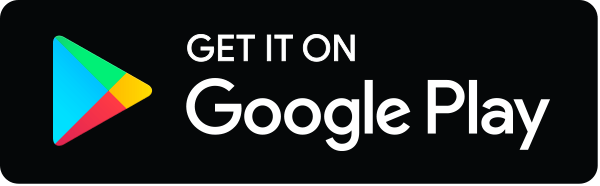
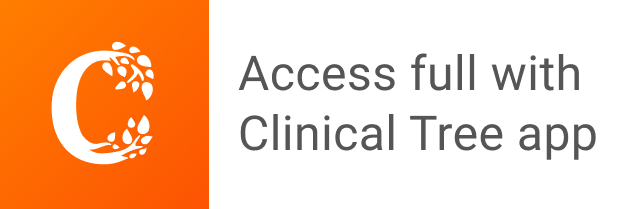