Nutrition
Sara E. Ramel
Michael K. Georgieff
The provision of nutrition to term and preterm newborn infants remains one of the most important aspects of neonatal care. With increasing survival rates among sick newborns, the nourishment of full-term and preterm infants has assumed an increasingly greater role in the neonatal intensive care unit (NICU) in the past 25 years, particularly since the link between nutritional management and neurodevelopment in preterm infants has become more obvious. Great strides have been made in understanding neonatal nutritional physiology and pathophysiology in these years, allowing physicians to more precisely estimate the nutritional needs of the infants in their care. Knowledge of newborn infants’ nutritional requirements and of their neurologic, gastrointestinal, and metabolic capabilities is a prerequisite to informed decision making about nutritional therapy in the nursery. It is also important to understand the tools available for assessment of neonatal nutritional status.
The goal of nutritional therapy in the term neonate is to ensure a successful growth transition from the fetal to the postnatal period. In the preterm infant, the goal has been to continue the process of intrauterine growth in the extrauterine environment until 40 weeks postconception and to foster catch-up growth and nutrient accretion in the postdischarge period. Until lately, the goal for the growing preterm infant has been to match the third-trimester intrauterine rates of weight gain, linear growth, and brain growth. Even when these rates are successfully attained, the body composition of the preterm infant raised in an extrauterine environment remains remarkably different than that of the same postconceptional-age fetus who has remained in utero (1,2). Current efforts are aimed at understanding the metabolic processes that determine the body composition of the preterm infant (3). The preterm infant is at risk for being well below the standard gestational growth curves at the time of hospital discharge because of nutrient deficits that have accrued during the prolonged period of neonatal illness (4). The effect of illness on neonatal metabolism and nutritional requirements is being recognized (3). Pathologic conditions such as bronchopulmonary dysplasia (BPD), congestive heart failure (CHF), acute respiratory distress, intrauterine growth restriction, and sepsis (and their treatments) have negative effects on neonatal energy and protein and mineral and vitamin requirements. These conditions may also affect the digestive and absorptive capacities of the neonate.
In this chapter, the nutritional requirements, digestive capabilities, and expected growth of term and preterm infants are reviewed. These factors will be addressed in the context of the three phases of neonatal nutritional development: transition, in-hospital growth, and postdischarge. The chapter also provides an overview of various nutrient delivery systems—both enteral and parenteral. Finally, the chapter covers techniques of nutritional assessment and offers suggestions for appropriate monitoring of neonatal nutritional status.
▪ NUTRITIONAL CAPABILITIES OF THE NEWBORN INFANT
Informed nutritional management of neonates depends on knowledge of their nutritional capabilities. The ability to suck and swallow a meal in a coordinated fashion and then process those nutrients for utilization by the body may be one of the most complex developmental tasks facing the newborn infant. Success depends on a certain amount of neurologic, digestive, absorptive, and metabolic maturity. The term infant is quite mature in these respects. However, the preterm infant’s physiology becomes progressively more mature as a function of increasing gestational age and experience. It is important to be able to distinguish between processes that are experience dependent and experience independent in order to set reasonable feeding plans and expectations.
Neurologic Maturity
The neurologically intact term infant is able to suck and swallow in a coordinated fashion within minutes of birth. In the preterm infant, the sucking reflex is strong at the limit of viability (23 weeks) and likely prior to that age (5). However, the ability to coordinate the suck reflex with swallowing to ensure that food is propelled into the gastrointestinal tract rather than the airway, matures at approximately 34 weeks’ gestation (5). The age at which the infant matures varies widely, and some preterm infants are able to suck and swallow in a coordinated manner by 32 weeks postconception. This coordinated suck-and-swallow reflex appears to be largely postconceptional-age mediated, although a Cochrane review confirmed that introduction of nonnutritive sucking (e.g., practice) prior to attempts at oral feeds reduces length of hospital stay even though it has no effect on age at full oral feeds (6).
Motility in the gastrointestinal tract is also dependent on neurologic maturation (7). For example, the esophagus shows a very discoordinated pattern of peristalsis at 24 weeks’ gestation, with weak peristaltic waves beginning sporadically and propagating either rostrally or caudally (7). By term, the pattern has matured into a coordinated pattern that propels food downward to the stomach (7). The lower esophageal sphincter of the very preterm infant is immature and provides little barrier to gastroesophageal reflux (GER). GER disease in the preterm infant can be associated with aspiration syndromes and feeding intolerance. At term, although GER remains demonstrable in most infants, it is generally not the potentially life-threatening problem seen at earlier gestational ages.
The stomach also undergoes maturation during the third trimester. The preterm infant’s stomach does not “wring” the contents in a coordinated fashion from the antrum to pylorus, frequently being subject to periods of antiperistalsis, which in turn leads to episodes of GER (8). Additionally, immaturity of pyloric function causes slower gastric emptying in the preterm infant (8). The small stomach capacity of the preterm infant mandates frequent small volume feedings. The prolonged gastric emptying time, however, causes retention of the food in the stomach and subsequent “preaspirates” prior to the next feeding. Since the presence of preaspirates may also signify an ileus associated with severe diseases such as necrotizing enterocolitis (NEC), feedings are often discontinued when preaspirates are obtained. The clinical appropriateness of routinely assessing preaspirates is questionable, since the positive predictive value for NEC is very low and the discontinuation or volume reduction of feeding with each preaspirate event adds to the cumulative nutrient deficit during hospitalization.
The maturation of small intestinal motility has been extensively studied (8). Orderly progression of peristaltic frequency, amplitude, and duration increases with gestational age. A 2-hour periodicity to peristalsis is found in very-low-birth-weight (VLBW) preterm infants (8), that may influence feeding schedules. The lack of a coordinated motility pattern in the very preterm infant makes it more likely that they will present with signs of feeding intolerance, usually marked by abdominal distention. When compared to adults, neonates retain food in their small intestine for proportionately longer periods and in their colon for shorter periods. The newborn’s ability to modulate stool water and electrolyte content is immature compared to adults.
Digestive and Absorptive Capabilities
The newborn infant does not have the capability to digest and absorb nutrients from a complex diet (5). Fortunately, the term human infant has a ready source of nutrition in the form of human milk (9). Human milk is remarkably adapted to fit the digestive capacities of the term newborn and to meet the infant’s nutritional needs for at least the first 6 months of life (see also Chapter 21) (9). Recent reviews emphasize the nutritional, immunologic and neuro-developmental value of feeding human milk to preterm infants (10). Human milk is now considered to be the gold standard for feeding the preterm infant. This represents a major shift in thinking in the last 25 years. Early in the history of modern neonatology, human milk was considered inadequate for preterm infants due to its low protein, energy, sodium, calcium, phosphorus, and iron contents. However, with fortification human milk has become the preferred diet because of its immunoprotective and brain maturational effects (10).
The functional immaturities of the gastrointestinal, hepatic, and renal systems in the newborn have an impact on the delivery of all classes of nutrients: macronutrients, minerals, trace elements, and vitamins.
Protein
Protein digestion begins in the stomach with the action of pepsin on the intact protein. Pepsin is activated by acid hydrolysis of its precursor molecule, pepsinogen. The newborn is capable of creating an acidic stomach environment by 1 week of age; thus, pepsin activation is thought to be intact. Dietary protein is then acted upon by pancreatic peptidases released into the duodenum. These enzymes include trypsin, chymotrypsin, carboxypeptidases A and B, and elastase and are amino acid selective with respect to cleavage sites, resulting in peptides of relatively small length. The peptides are subsequently cleaved once more by peptidases located in the intestinal mucosal cells, absorbed as amino acids or dipeptides, and transported to the liver. Protein digestion and absorption in adults is very efficient; up to 95% of a protein load can be fully digested. Although term and preterm infants have relatively low concentrations of chymotrypsin, the carboxypeptidases, and elastase (11), they nevertheless achieve more than 80% protein digestion.
Fat
The efficiency of fat digestion in the neonate has been a controversial topic. Whereas adults will absorb close to 95% of a fat meal and term infants absorb 85% to 90%, early studies indicated that preterm infants absorb as little as 50%, depending on the type of fat presented to them (12). These studies did not take into account lipases that are found in nonpasteurized mother’s milk (but not in infant formula) that support fat absorption at a greater than 90% rate (12). The low rate of fat digestion in the preterm infants fed with infant formula led to modification of fat blends in the formulas.
Fat digestion in the neonate begins in the stomach with the action of a lipase secreted in the mouth (lingual lipase) or by the gastric mucosa (gastric lipase) (12). The two lipases are identical, function ideally at acid pH, work primarily on medium-chain triglycerides (MCT), and do not require bile salts. Oral and gastric lipase may be responsible for up to 50% of fat digestion in the newborn (12). Infants fed with unpasteurized human milk have the additional benefit of a lipase secreted into the milk by the mother (12). Breast milk lipase is found in all carnivores (but not herbivores) and functions more like pancreatic or intestinal lipases found in adults. It is destroyed by the pasteurization process. Practically speaking, this means that pasteurized donor human milk will be more poorly absorbed than fresh/frozen own mother’s milk. Breast milk lipase works primarily on long-chain triglycerides at a neutral pH, as is found in the intestine, and requires bile salts. Breast milk lipase may be responsible for the digestion of up to 20% of dietary fat (12). The combination of gastric, lingual, and breast milk lipases are referred to as the “compensatory lipases” of the newborn and function in place of pancreatic and intestinal lipases seen in more mature humans (12).
Long-chain fatty acids are dependent on bile salts for proper micellization and uptake into the intestinal lymphatics. From there, the micelles are carried to the venous system via the thoracic duct, ultimately destined for the liver. Medium-chain fatty acids do not require micellization and can be directly absorbed into the blood stream. The bile acid, and hence bile salt, pools of the preterm newborn are low, thus potentially restricting the fat absorption capacity of the infant (13). Prenatal administration of glucocorticoids to the mother can mature the fetal bile salt pool in the preterm infant less than 34 weeks’ gestation to the level of the term infant (13). Without such priming, however, the preterm infant has significant impairment of fat absorption (including fat-soluble vitamins) prior to 34 weeks’ gestation. The fat blend in preterm infant formulas designed for infants less than 34 weeks’ gestation is modified to optimize fat absorption. These formulas contain a higher percentage of MCT and higher vitamin A, D, and E levels than formulas manufactured for term infants.
Carbohydrate
Like fats, carbohydrates can present a significant digestive challenge (14). The neonate has a limited ability to digest complex carbohydrates because of relatively small amounts of pancreatic amylase. Thus, beikost in the form of cereal rarely makes up a significant portion of the infant’s diet until after 4 months of age. Term and preterm newborns readily use glucose, which can be delivered either parenterally or enterally. Intestinal glucose uptake is seen as early as 10 weeks’ gestation, long before the fetus is viable (14). However, provision of all carbohydrate calories as glucose would result in the neonatal gut being exposed to a hyperosmolar solution with a potential to cause mucosal damage.
The primary carbohydrate found in mammalian milk is the disaccharide lactose. Like other disaccharides (sucrose, maltose, isomaltose), enzymatic cleavage by a disaccharidase must occur before the monosaccharides can be absorbed. In the case of lactose, glucose and galactose are produced by the action of lactase. The disaccharidases sucrase and maltase appear very early in gestation and appear to be inducible enzymes. In contrast, lactase begins to appear at 24 weeks’ gestation and rises in concentration slowly until term (14). It does not appear to be a particularly inducible enzyme with feeding of lactose (14). The preterm infant can present as functionally lactose intolerant and will have typical symptoms of gas formation, diarrhea, and acidic stools characteristic of lactose malabsorption when fed high doses of lactose. Preterm infant formulas have lower lactose contents than term formulas for this reason.
Up to 60% of carbohydrate calories in preterm infant formulas are derived from linear glucose polymers, which produce a lower osmolar load than the equivalent number of individual glucose molecules. The enzyme required to digest glucose polymers (glucoamylase) is present from 24 weeks’ gestation (14). The lower lactose content is also present in the premature discharge formulas, although it is likely that the preterm infant is fully mature with respect to lactose absorption at the time of discharge (14).
▪ NUTRIENT REQUIREMENTS FOR TERM AND PRETERM INFANTS
Estimation of nutrient requirements is an inexact process, particularly when the goal is unclear. To date, the goal has been to achieve the same growth rates and body composition as the “reference” infant. For term infants, the “gold standard” remains the healthy breastfed infant, whose ideal growth was clarified by the World Health Organization (WHO) with the release of standard growth charts (15). Human milk composition varies greatly among mothers, and the length of time that it remains sufficient for all the
nutrient needs of the infant is not uniform. Breastfed infants may have lower iron stores (16) and be at greater risk for vitamin D deficiency than formula-fed infants (17).
nutrient needs of the infant is not uniform. Breastfed infants may have lower iron stores (16) and be at greater risk for vitamin D deficiency than formula-fed infants (17).
Determining the ideal growth for the infant born before term is far more problematic. Indeed, the ideal growth rate and body composition of the “healthy” preterm infant remain unknown and are likely to be different from his or her gestationally age-matched fetal counterpart. Until recently, the daily and weekly accretion rates of various nutrients in the preterm infant have been modeled on in utero accretion rates of these nutrients in gestationally age-matched fetuses. The “reference fetus” described by Widdowson and again by Ziegler has served as the benchmark by which neonatal nutritionists judge fetal growth and body composition (2). Nevertheless, energy requirements are likely to be different in a 28-week gestational-age newborn exposed to the thermal stresses of extrauterine life than in a 28-week fetus comfortably surrounded by amniotic fluid.
The rates of weight gain, linear growth, and head growth between the ages of 24 and 36 weeks’ gestation can be calculated from the standard growth curves generated from infants born prematurely (18). It must be recognized that the data used to generate these plots are necessarily cross-sectional and thus need smoothing to create the resemblance of a curve. Additionally, since premature birth is an abnormal event and up to 30% of VLBW infants are small for dates (most likely as a result of the pregnancy failing over time), the reliability of using newborn data to assess the growth velocity of healthy fetuses is suspect. Nevertheless, these curves are used extensively as guideposts for neonatal growth of the preterm infant. On average, these curves predict that the preterm infant should gain 15 to 18 g/kg body weight each day, grow 1 cm/wk linearly, and demonstrate 1 cm/wk of head growth. However, greater weight gain velocity (20 to 30 g/kg/d) may be necessary for preterm infants to achieve normal birth weight z-scores by term.
The nutrient requirements of term and preterm infants can be calculated based on fetal reference figures, balance studies, serum nutrient values, or a combination of these.
Energy Requirements
Energy requirements must take into account the amount and caloric density of the solution ingested, the route of administration (enteral vs. parenteral), the amount lost in stool or urine, and the energy requirements in the body (e.g., basal metabolic rate, cost of growth, energy cost of food processing by the body). Many of these parameters are now measurable, and reasonable estimates of energy requirements to maintain optimal weight gain and linear growth velocities can be made for both term and preterm infants.
Energy is predominantly derived from carbohydrates and fat in the diet, which provide 4 and 9 kcal/g, respectively. The infant fed human milk receives calories predominantly from fat, whereas the formula-fed infant receives calories more evenly distributed between fat and carbohydrate (19). The calories derived from these sources are used first to maintain the total energy need of the infant, which consists of the basal metabolic rate, the thermic effect of feeding, and physical activity. Energy intake beyond this baseline is stored and recorded as weight gain. Protein is not normally utilized as an energy source, unless the total energy intake is less than the total energy expenditure of the infant. In those cases, certain amino acids can be deaminated and shunted into the gluconeogenic pathways to provide approximately 4 kcal/g of protein (20). This emphasis on maintaining glucose delivery is a consequence of the high metabolic rate of the neonatal brain, which relies primarily on glucose as its energy substrate.
Energy requirements can be affected by numerous factors, including the route of delivery and the disease state. Energy requirements are approximately 10% lower when infants are fed parenterally as opposed to enterally because no energy is excreted in the stool. Diseases that increase energy needs include CHF (21), BPD (22), acute respiratory disease (23), and overwhelming sepsis (24). Diseases that decrease energy needs include hypoxic-ischemic encephalopathy and degenerative neurologic conditions in which there is paucity of physical movement. Therapies can also alter energy requirements. Catecholamine infusions and caffeine can increase energy expenditure (25), while mechanical ventilation, extracorporeal membrane oxygenation (ECMO), and total body cooling can reduce it.
Term Infants
Healthy breastfed term infants show adequate growth on as little as 85 to 100 kcal/kg body weight per day during the first 4 months of life (26). Formula-fed infants have higher energy requirements (100 to 110 kcal/kg), most likely as a result of a lower efficiency of digestion and absorption of fat (12). See section on “Digestive and Absorptive Capabilities”, above.
Preterm Infants
Preterm infants have higher energy requirements than term infants because of a higher resting energy expenditure and greater stool losses as a result of immature absorptive capacities (see “Digestive and Absorptive Capabilities” section). Whereas the resting energy expenditure of the term infant is 45 to 50 kcal/kg/d, the preterm infant less than 34 weeks’ gestation consumes 50 to 60 kcal/kg/d (27). Stool losses vary between 10% and 40% of intake, depending on the diet. For example, a diet in which the carbohydrate is 100% lactose and the fats are predominantly long-chain triglycerides will promote more stool losses because of the low levels of lactase and the small bile salt pools in the premature infant. Replacement of up to 50% of the lactose with glucose polymers and between 10% and 40% of the fat with MCT appears to reduce malabsorption to approximately 10% (28). The preterm infant will need an additional 50 to 60 kcal/kg/d beyond the daily energy expenditure and the loss of energy in the stool to maintain weight gain along the intrauterine growth curve (15 to 18 g/kg/d). Thus, barring any excess needs from diseases that increase oxygen consumption or from malabsorption, the preterm infant will gain weight parallel to the growth curve on approximately 120 kcal/kg/d. Assuming that 2.5 kcal are needed to achieve 1 g of weight gain, the upward adjustment to a greater weight gain velocity of 20 to 30g/kg/d would require feeding an additional 10 to 15 kcal/kg body weight per day to the preterm infant. Thus, it is likely that energy intake on the order of 130 to 135 kcal/kg/d is a more reasonable target for the premature infant than the previous recommended intake of 120 kcal/kg/d, especially given that most infants will have fallen away from the curve during their period of illness and will have accrued deficits that need to be made up in addition to standard expected weight gain (4). Many are hesitant to increase caloric intake due to concerns of increased adiposity and long-term metabolic risk; however, to date, this has not been verified in the literature. Increasing caloric intake (as high as 150 kcal/kg/d) increases fat-free mass gains and linear growth without increasing fat mass (29). More research is needed to further evaluate the optimal weight gain velocity, taking into account both long-term metabolic and neurodevelopmental outcomes. Additionally, all growth parameters (weight, length, head circumference, body composition) must be taken into account when evaluating the energy requirements of preterm infants.
Energy Sources
Carbohydrates
Newborn infants are highly dependent on a source of glucose for normal brain metabolism (30). The primary source of glucose in the term infant is lactose in human milk and cow’s milk formulas. Soy-based formulas provide glucose from the metabolism of dietary sucrose or glucose polymers. Preterm infants also receive glucose, initially as dextrose in parenteral solutions, but subsequently enterally from lactose or glucose polymers. Galactose is also important to the newborn, as it is needed for glycogen storage. The newborn infant
typically utilizes between 4 and 8 mg/kg/min of glucose (30). This figure is commonly used as the glucose infusion rate for parenteral nutrition. Because of their low glycogen stores and poorer gluconeogenic capacities, preterm infants are more prone to hypoglycemia than term infants. Higher rates of glucose delivery (up to 15 mg/kg/min) may be required in growth-retarded infants and in infants of diabetic mothers to maintain normal glucose concentrations.
typically utilizes between 4 and 8 mg/kg/min of glucose (30). This figure is commonly used as the glucose infusion rate for parenteral nutrition. Because of their low glycogen stores and poorer gluconeogenic capacities, preterm infants are more prone to hypoglycemia than term infants. Higher rates of glucose delivery (up to 15 mg/kg/min) may be required in growth-retarded infants and in infants of diabetic mothers to maintain normal glucose concentrations.
Intravenous dextrose infusion rates up to 12.5 mg/kg/min are commonly used in preterm infants to promote catch-up weight gain. Beyond this rate, a cost/benefit analysis must be made. Although faster rates of weight gain can be achieved on higher glucose infusion rates (especially if the serum glucose is controlled with exogenous insulin infusion) (31), a higher metabolic rate and a shift in the respiratory quotient will also occur. Thus, a higher oxygen consumption rate coupled with proportionately more carbon dioxide generated by the cells may significantly affect serum carbon dioxide and ventilatory requirements. Moreover, the increased “growth rates” demonstrated with high glucose infusion rates are a result of fatty weight gain, without any increase in linear or brain growth (31). In addition to the short-term concerns of increased adiposity, there are concerns regarding the long-term effects of early hyperglycemia, with worsened growth and neurodevelopmental outcomes in those infants with more days of hyperglycemia (32). Therefore, the risks and benefits of aggressive support with high (>12.5) glucose infusion rates must be carefully considered.
Fats
Lipids are a major energy source for neonates. Certain fatty acids, such as linoleic (omega-6, 18:2) and linolenic (omega-3, 18:3), are essential in the diet, and their absence will produce deficiency syndromes. Essential fatty acid concentrations decline rapidly within 1 week of discontinuing lipid intake. Infants receiving parenteral nutrition or on a fat-restricted enteral diet require at least 0.5 mg/kg/d of an intravenous fat blend containing these fatty acids at least three times per week to prevent severe essential fatty acid deficiency. The American Academy of Pediatrics (AAP) has recommended that at least 3% of total energy intake in infants should be in the form of linoleic acid (33).
Daily fat intake varies greatly based on the method of delivery (enteral vs. parenteral) and the dietary source (human milk vs. formula). Enterally fed term infants consume approximately 5 to 6 g/kg/d of fat, whereas parenterally fed infants rarely receive greater than 3.5 g/kg/d. Infants receiving human milk (especially human milk expressed by mothers who have delivered preterm) may receive up to 7 g/kg/d.
Infants fed human milk receive a unique blend of fats that cannot be precisely replicated in infant formulas. Cow’s milk fat is generally not well tolerated by newborn infants, requiring formula manufacturers to use vegetable oils as substitutes. The spectrum of fatty acids found in infant formula are distinctly different from human milk fats.
The role of omega fatty acids such as docosahexaenoic acid (DHA) and arachidonic acid (AA) in the infant diet continues to be a subject of intense research. These fatty acids are products of an elongation pathway from linoleic acid and are important in cell membrane structure, in cell-signaling cascades and in myelination (34). The synthetic pathways are usually immature in preterm infants and for some undetermined period of time after birth in term infants (34). Levels of DHA and AA decline immediately after birth (35). Sources of these fatty acids include the placenta and human milk. In contrast, cow’s milk fat and vegetable oil do not contain these compounds. Because the preterm infant may be less capable of synthesizing the compounds and would have received them in utero, the European Society for Pediatric Gastroenterology and Nutrition has recommended that a source of these fatty acids be added to preterm infant formula (36). Evidence of their efficacy includes studies that demonstrate better visual acuity, more mature electroretinograms, and short-term gains in general neurodevelopment in supplemented infants (37). Studies of the longer-term growth and developmental outcomes of infants who have been supplemented with these fatty acids suggest continued positive effects on the visual system at 1 year of age (37). It remains unclear whether potential early neurodevelopmental gains are sustained beyond the 1st year. Cochrane reviews assessing whether supplementation of long-chain polyunsaturated fatty acid (LC-PUFA) in infant formula improves neurodevelopment in term (38) and preterm (39) infants have been performed multiple times over the past 12 years. The most recent, which included 25 high-quality trials in term infants and 13 high-quality trials in preterm infants, concluded that the studies do not support a positive long-term neurodevelopmental effect following supplementation via infant formula in the neonatal period (38,39). On the other hand, no adverse effects were noted. An important consideration in the addition of any one compound to an infant’s diet is whether the component exerts its nutritional effect individually or in consort with other compounds. Based on their “generally recognized as safe” (GRAS) status, DHA and AA have been added to term, preterm hospital, and preterm discharge formulas by the major formula manufacturers.
The lack of a consistent neurodevelopmental effect may be as a result of suboptimal dosing of the compounds or may represent a washout of early effects over time. However, neurodevelopment is not the only outcome of relevance to these compounds. Martin et al., report that even small reductions in DHA and AA are associated with increased chronic lung disease and late-onset sepsis in preterm neonates (35).
Carnitine is another compound involved in fat metabolism in the neonate. Although carnitine deficiency is rare in the enterally fed infant because of high levels in human milk and supplementation of formulas, it remains a concern in infants receiving long-term exclusive parenteral nutrition in which carnitine has not been supplemented. Carnitine supplements can be added to total parenteral nutrition (TPN) and are recommended in infants who receive TPN for greater than 3 weeks. However, routine carnitine supplementation of preterm infants from birth has no positive effect on growth, apnea, or length of hospital stay and thus is not indicated (40).
Protein Requirements
Protein requirements in humans are determined by a number of factors including protein quality and quantity, the amount of energy delivered, and the protein nutritional status of the subject. The latter is influenced by the degree of previous malnutrition, by the rate of catch-up growth, and potentially by inflammatory processes. The sick newborn infant is exposed to many of these influences. Additionally, they have a high basal requirement for protein accretion based on in utero nitrogen accretion rates (2). Adequate energy intake is important to promote optimal protein utilization, with a nonprotein calorie-to-gram nitrogen ratio of 200:1 considered ideal. Protein intake in the neonate has previously been limited to about 4 g/kg/d because of concerns regarding the inability of the immature kidney to excrete titratable acid, blood urea nitrogen (BUN), and ammonium ion. However, more recent data have confirmed that levels of 4.5 to 5 g/kg/d are tolerated without increased BUN levels or metabolic acidosis (41).
Protein requirements, in general, and branched-chain amino acid needs, in particular, are increased in adults with physiologic instability as a result of septic or surgical illness. The possibility that similar changes might occur in sick neonates has been investigated (23,24). Neither acute respiratory disease nor sepsis nor surgical ligation of the patent ductus arteriosus (PDA) results in increased protein requirements (23,24). At this time, increasing protein delivery routinely on the basis of illness or physiologic instability is not recommended. Conversely, practitioners frequently limit nutrient delivery during illness out of concern that high loads may be metabolically taxing. Up to 3 g of protein per kg body weight can
safely be administered daily to sick preterm infants beginning in the first 24 hours after birth (42), and currently recommendations are to advance to at least 4 g/kg daily as tolerated.
safely be administered daily to sick preterm infants beginning in the first 24 hours after birth (42), and currently recommendations are to advance to at least 4 g/kg daily as tolerated.
Term Infants
The full-term breastfed infant grows adequately and maintains normal serum and somatic (i.e., muscle) protein status on as little as 1.5 g/kg/d of protein. Although the protein content is low (1.1%), the quality of human milk protein is excellent because the spectrum of amino acids provides a unique “match” for the amino acid needs of the newborn. The protein content is predominantly lactalbumin, as opposed to casein, which makes for smaller curds and easier digestibility. Additionally, human milk is replete with nondietary nitrogen sources including nucleotides, which may enhance the immune system; immunoglobulins and other antimicrobial factors, which help protect the gut epithelium; growth factors, which stimulate intestinal growth; and enzymes (e.g., lipases), which aid digestion (9).
The term infant fed a cow’s milk or soy-based infant formula requires a greater protein delivery rate, most likely to compensate for the less-than-ideal protein quality. Thus, the infant on cow’s milk formula typically requires 2.14 g/kg/d and the infant on soy formula up to 2.7 g/kg/d of protein (19). Cow’s milk protein is predominantly casein, although a number of cow’s milk-based formulas are modified to be whey predominant. The soy formulas also promote adequate growth of lean body mass. However, these formulas contain a smaller percentage of available nitrogen as essential or semiessential amino acids (19).
Protein can also be delivered to the term infant by way of protein hydrolysate or individual amino acid formulas. These formulas are specifically designed to decrease the exposure of the infant to potentially antigenic cow’s or soy milk proteins. By hydrolyzing the cow’s milk-based protein such that greater than 90% of the proteins have a molecular weight of 1,250 Da or less, allergic disease as a result of cow’s milk allergy can be treated or potentially prophylaxed. These formulas provide approximately 2.8 g/kg/d of protein at an energy delivery of 100 kcal/kg/d.
Preterm Infants
Recommendations for protein intake in preterm infants follow many of the same parameters as in term infants. However, the needs of the preterm infant appear to be greater than the term infant. Preterm infants require protein initiation immediately after delivery to prevent loss of endogenous stores (43). In addition, preterm infants build up early deficits in protein due to illness, or transition to enteral feeds, and therefore likely require higher amounts later to correct for early losses (43). Studies of the protein requirements and distribution (synthesis vs. breakdown) in extremely low-birth-weight (ELBW) infants demonstrate that an average of 3.2 g/kg of protein is necessary to counter the negative nitrogen balance of neonatal illness and to match the expected in utero protein accretion rate (43). Growth and developmental outcomes are improved when protein intakes closer to 4 to 5 g/kg/d are established early and maintained throughout hospitalization. Olsen et al. (41) have shown that infants fed 4.6 to 5.5 g/kg/d have less decline in length z-scores. Long-term growth (weight, length, and fat-free mass gains) and developmental outcomes have also been shown to improve with increased protein intake (approximately 4.5 g/kg/d) (3). Besides total protein delivery, studies have considered which amino acids may limit protein accretion in the preterm infant. The terms “essential” and “nonessential” amino acids have been replaced in the neonatal lexicon by “indispensable” or “limiting” and “dispensable” because they are more descriptive of the effects of amino acids on protein metabolism. Threonine and lysine are clearly indispensable because they cannot be synthesized de novo from products of carbon intermediary metabolism (44).
Finally, illnesses or medications that increase protein turnover or muscle breakdown will have an influence on protein delivery. Van Goudoever et al. (45) demonstrated that the steroids used for the treatment of BPD cause negative nitrogen balance by increasing the rate of protein breakdown but have little effect on protein synthesis. More recently, the influence of illness and inflammation on linear growth and fat-free mass gains (markers of protein accretion) has been recognized and documented (3). Increased markers of inflammation and illness continue to negatively influence linear growth out to 24 months corrected age for prematurity (3).
Many preterm infants receive protein initially as part of a regimen of parenteral nutrition. Intravenous amino acid solutions have advanced to the point of being specifically formulated for preterm infants. These amino acid solutions are designed to normalize the plasma amino acid profile of the healthy infant, promoting levels similar to those of a 1-month-old breastfed infant. Infants receiving early amino acid solutions have improved and positive nitrogen balance and better developmental scores at 18 months (3,42,44). For these reasons, current recommendations for amino acid delivery are to begin at 3 g/kg/d immediately after birth and increase by 0.5 to 1 g/kg/d to approximately 4 g/kg/d (42,44).
Preterm infants who begin enteral feeds in the first days after birth will be relatively protein restricted because they are typically given human milk or low amounts of preterm infant formula. Although human milk from mothers delivering preterm is initially higher in protein compared to term human milk (10), the content is still relatively low and rapidly decreases postnatally to term levels. In addition, the infant initially will receive low volumes while slowly advancing enteral feeds. Fortification with a human milk fortifier assists with this low-protein issue, however, still may not be enough. More recently, individualized fortification with fortifiers and additional protein supplementation has been recommended (46). Infants receiving this individualized fortification have been shown to have improved weight and head circumference gains (46).
Mineral and Element Requirements
Mineral requirements in newborns are influenced by the immature status of the kidney, the degree of prematurity, and medications that affect mineral metabolism (33). In general, preterm infants require higher amounts of minerals than term infants. The daily needs of some minerals (e.g., sodium, potassium chloride) are determined by measuring serum levels, although others (e.g., calcium, phosphorus) are estimated from in utero accretion rates (2). A more comprehensive discussion of these needs can be found in other chapters in this volume (e.g., see Chapters 19 and 33).
Iron
The majority of total body iron found in the term infant is accreted during the third trimester (2). The fetus maintains a constant total body iron content of 75 mg/kg during the last trimester, increasing from 35 to 40 mg at 24 weeks’ gestation to 225 mg at term. Preterm delivery results in disruption of this process, and premature infants, including the late preterm, are therefore born with lower iron stores than term infants (47). Small-for-dates infants are frequently born with low iron stores, presumably because of decreased placental iron transport (47). Infants of diabetic mothers are born with low stores because much of the fetal iron is in the expanded red cell mass (47). These infants also appear to be low in total body iron, most likely as a result of altered transport of iron by the diabetic placenta, such that the increased iron need of the infants of diabetic mothers exceeds the placental transport capacity (47). Table 20.1 outlines the iron requirements for newborns of various birth weight and gestational age categories.
Term Infants
The breastfed appropriate-for-dates newborn infant who benefitted from delayed cord clamping and grows at a rate prescribed by the WHO standard curve has sufficient total body iron to remain nonanemic for 6 months (48). The small-for-dates infant has closer to a 2-month reserve and is at high risk for postnatal iron deficiency
if not adequately supplemented with dietary iron. The estimated daily iron requirement for the formula-fed term infant is 1 mg/kg/d (49) (Table 20.1) (see also Chapter 43).
if not adequately supplemented with dietary iron. The estimated daily iron requirement for the formula-fed term infant is 1 mg/kg/d (49) (Table 20.1) (see also Chapter 43).
TABLE 20.1 Iron Dosing for Neonates Based on Gestational, Size for Dates and Diet | ||||||||||||||||||||||||||||
---|---|---|---|---|---|---|---|---|---|---|---|---|---|---|---|---|---|---|---|---|---|---|---|---|---|---|---|---|
|
The major dietary source of iron for the healthy, term infant is human milk or infant formula fortified with iron (49). Although human milk has a low iron content (0.3 mg/L) compared to either iron-fortified infant formula (10 to 12 mg/L) or “low-iron” formula (4.5 mg/L), the iron is more bioavailable (49). Infants fed formulas containing either 4 or 7 mg/L remain iron sufficient (50).
Preterm Infants
The preterm infant that is not growth restricted as a fetus begins extrauterine life with the same iron stores per kilogram body weight as the term infant (approximately 12 mg/kg). Serum ferritin concentrations, which reflect iron stores, are slightly lower in preterm infants (47). However, the preterm infant is exposed to several stressors that perturb iron balance; with the result that by the time of hospital discharge, the infant may be iron deficient or iron overloaded. The range of iron status of the preterm infant at 40 weeks postconception appears to be far wider than the term infant, although systematic studies are lacking.
The preterm infant frequently goes into negative iron balance because of blood lost during phlebotomy coupled with a rapid growth rate (and expansion of the red cell mass) during the convalescent period (see also Chapter 43). The daily enteral iron requirement for the preterm infant who does not receive recombinant erythropoietin is 2 to 4 mg/kg/d, with the greater requirement for the more preterm infant (51). Infants who receive recombinant erythropoietin require at least 6 mg/kg/d of iron (Table 20.1) (51).
The issue of when to begin iron supplementation in the preterm infant is controversial. Iron is necessary for normal growth and development of all tissues, including the brain. A rich literature supports the hypothesis that early iron deficiency results in neuro-developmental sequelae at the time of the deficiency and persists well after iron has been repleted (52). Iron deficiency in preterm infants slows nerve conduction (53). Nevertheless, iron is also a potent oxidant stressor since it catalyzes the Fenton reaction to produce reactive oxygen species. Since preterm infants have immature antioxidant systems, there is concern that free iron (i.e., in excess of the total iron-binding capacity) can exacerbate diseases that may be related etiologically to oxidative stress, including BPD, NEC, neuronal injury, and retinopathy of prematurity (ROP) (54). Infants who receive parenteral iron are at risk for having free circulating iron and increased markers of oxidative stress (55). Because of these concerns, parenteral iron should be used very sparingly. Conversely, enteral iron doses of up to 18 mg/kg daily have been given without sequelae (56). Since infants are born with adequate iron stores, there is no need to begin iron supplementation in a sick, nongrowing preterm infant. Therefore, enteral iron supplementation should not be started before 2 weeks postnatal age (33). Conversely, delaying iron supplementation until after 2 months confers a high risk of iron deficiency in the postdischarge period.
Human milk should be used whenever possible in preterm infants. However, because of the low iron content of human milk and the rapid growth rate of these infants, additional iron supplementation is required. Additionally, those who receive recombinant human erythropoietin should be supplemented earlier with iron sulfate to have an adequate erythropoietic response. Preterm infant formulas are iron fortified and should provide adequate amounts to the larger preterm infant. However, preterm infants less than 30 weeks’ gestation may well need enteral iron supplementation in addition to their preterm formula or fortified human milk to bring their total dose closer to 4 mg/kg/d. Those with serum ferritin concentrations below 75 µg/L may require additional iron, as that level in newborns has been associated with neurologic sequelae (53).
Trace Elements
Ten trace elements are nutritionally essential for the human: zinc, copper, selenium, chromium, manganese, molybdenum, cobalt, fluoride, iodine, and iron (57). Excellent reviews of trace element requirements in newborns exist in the literature (57). Most trace elements are accreted during the last trimester. Thus, the appropriate for gestational age (AGA) term infant is fully replete and needs modest dietary intake of these elements. Both human milk and infant formula ensure adequate intakes. The preterm infant or the term infant on prolonged TPN would rapidly go into negative balance of any of these elements if not provided with an exogenous source. Infants should receive neonatal trace elements while on TPN. Preterm infant formulas and preterm human milk appear to supply adequate amounts of trace elements to the enterally fed premature infant (57).
Selenium is a potent antioxidant. Preterm infants have lower selenium stores than term infants (57
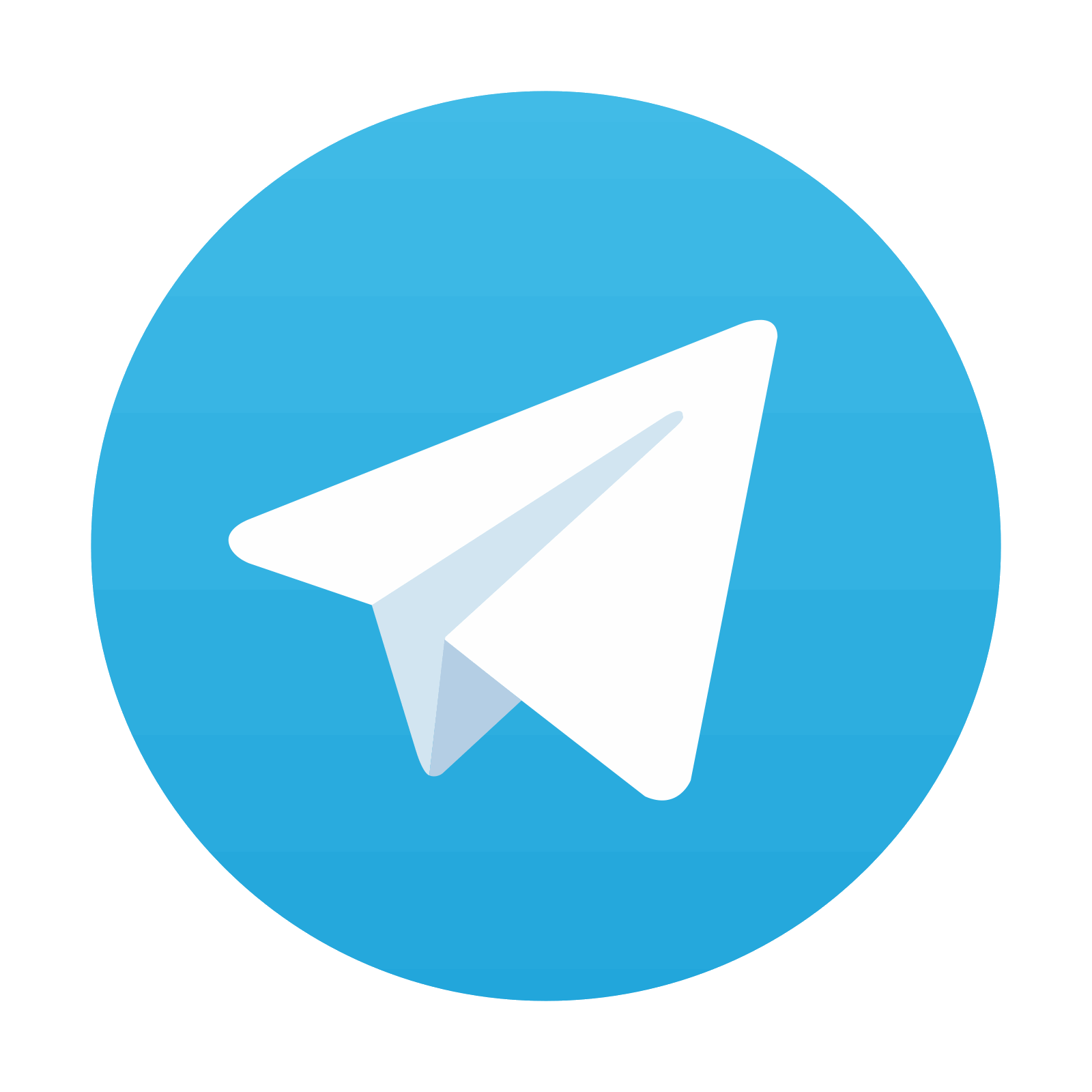
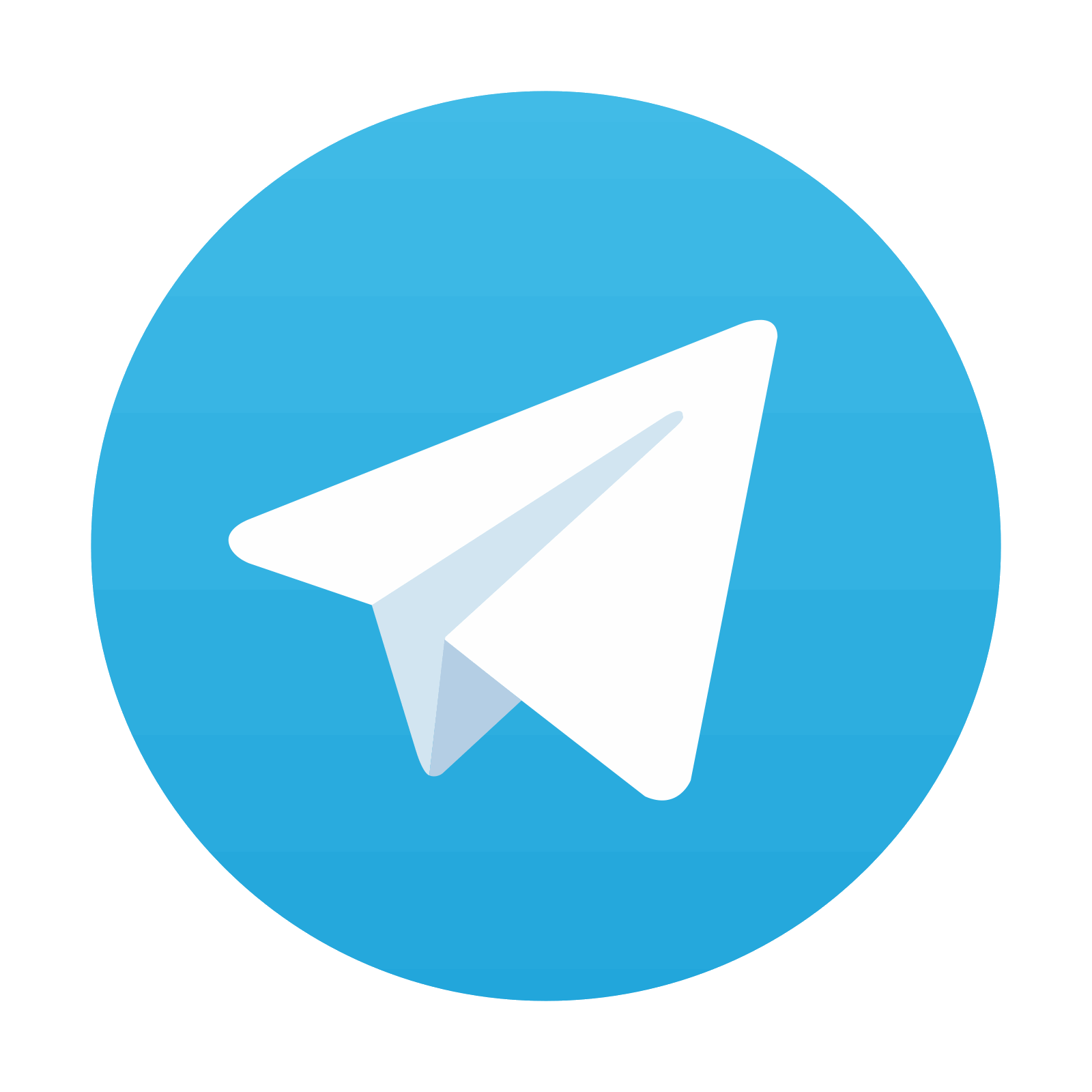
Stay updated, free articles. Join our Telegram channel
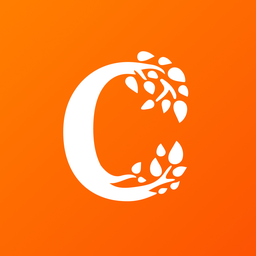
Full access? Get Clinical Tree
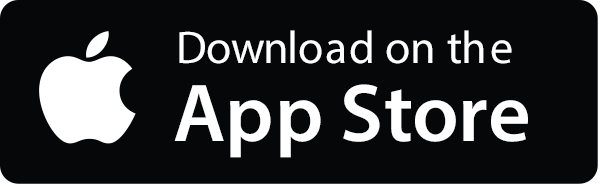
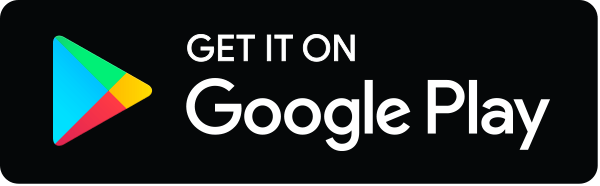
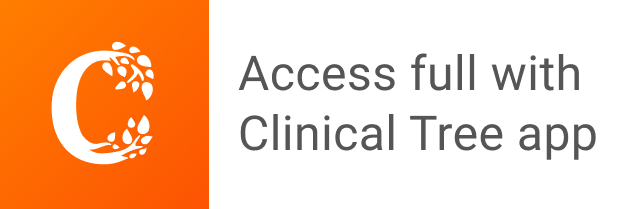