Mediastinum and Pleura
Bradley M. Rodgers
Eugene D. McGahren III
Department of Surgery, University of Virginia Health System, Charlottesville, Virginia 22906.
Department of Surgery, University of Virginia Health System, Charlottesville, Virginia 22906.
THORACIC EMBRYOLOGY
Most of the important embryologic events in the development of the thorax are completed by the eighth or ninth week of gestation. The thoracic cavity fully separates from the abdominal cavity by closure of the pleuroperitoneal folds of the diaphragm during the eighth week of gestation (1). Failure of complete closure results in congenital diaphragmatic hernia. Diaphragmatic muscularization occurs principally by the ingrowth of muscle from the lateral chest wall. Arrest of this process may produce anomalies such as congenital eventration of the diaphragm. The paired pleural cavities begin to form by the invagination of the lung buds into the paired pericardioperitoneal canals, connecting the primitive pericardial and peritoneal cavities. The pleural cavities are isolated by the development of the pleuropericardial and pleuroperitoneal membranes, a process completed by the sixth week of gestation (2) (Fig. 60-1). During the sixth week of development, the lateral thoracic wall begins to form by extensions of the lateral vertebral processes, which form the ribs. During this same interval, the sternum forms anteriorly as a pair of bands of condensed mesenchyme. By the seventh week, these sternal bands begin to fuse cranially, displacing the heart caudally. Fusion of the sternum and its junction with the developing ribs is complete by the ninth week of gestation (3). Abnormalities of development at this stage may result in sternal clefts and complex abnormalities of chest wall development, such as Poland syndrome.
During the third week of gestation, the laryngotracheal groove forms at the cranial end of the foregut, giving rise to the primitive trachea. At the same time, proliferation of mesenchyme of the foregut mesentery leads to the development of the cartilage, muscle, and connective tissue of the lungs. By the fourth week of gestation, the elongating trachea has bifurcated into right and left mainstem bronchi, and by the sixteenth week, segmental bronchi have begun to form (4). Abnormalities of this segmentation may lead to forms of pulmonary agenesis or tracheal branching abnormalities, such as bronchogenic cysts. The close timing of parallel embryologic events occurring in the trachea and the esophagus during this interval often leads to complementary abnormalities in these two structures, such as tracheoesophageal fistula.
The pulmonary arteries begin as a capillary network extending caudally from the aortic sac. By the sixth week of gestation, there are bilateral sixth aortic arches carrying blood to the developing pulmonary mesenchyme. By the seventh week the right arch has involuted, leaving the left sixth arch, which is the primordium of the main pulmonary artery. Abnormalities occurring during this interval may lead to defects of pulmonary vascular development, such as pulmonary sequestration. The details of bronchopulmonary development are outlined in greater detail in Chapter 61.
Between the sixth and eighth weeks of gestation, the jugular lymphatic sacs are connected with the cisterna chyli through bilateral systems of thoracic lymphatic channels, which are interconnected by numerous branches (5). The inferior portion of the right channel and the superior portion of the left, together with a diagonal channel at the level of the fourth thoracic vertebra, persist to form the definitive thoracic duct by the ninth week of gestation. Abnormalities of this process explain the high incidence of anatomic variation of the thoracic duct system. The thymus arises from the third pharyngeal pouch in the fifth week of gestation. Between the seventh and eighth week, the thymus elongates caudally until, by the end of the eighth week, the left and right primordia have fused at the level of the aortic arch (6). By the end of the ninth week, the mesenchyme of the thymus begins to be populated with lymphoid cells, probably of systemic origin.
POSTNATAL THORACIC DEVELOPMENT
Structurally, the chest and mediastinum are almost fully developed at the time of birth; however, a few changes take place later. The first and most dramatic change is the shift from fetal to adult circulation. During the infant’s first breaths, the pulmonary vascular resistance falls dramatically. This allows the pulmonary vasculature to accept the entire cardiac output, thereby facilitating closure of various fetal shunts, particularly the foramen ovale and the ductus arteriosus. The ductus functionally closes shortly after birth through contraction of its muscular wall; however, its anatomic closure is accomplished over 1 to 3 months by proliferation of the intimal layer (7,8).
The lungs continue their development after birth in a more subtle manner. Initially, in the postnatal period, the number of immature alveoli increases, and these alveoli are able to generate new immature alveoli, which subsequently enlarge into mature alveoli. Consequently, the area of the air–blood interface continues to increase as the alveoli and capillaries multiply. These changes continue through at least the eighth postnatal year. Only about 50 million, or one-sixth of the adult number of alveoli, are present at birth (9). The structural integrity of the airway improves after birth as the flexible cartilage of the infant’s larynx and trachea becomes more rigid.
ANATOMY
Fully developed, the mediastinum and the pleural spaces represent a collection of complex organs interacting in a constant and literally fluid manner. The mediastinum represents the central portion of the thoracic cavity. Its boundaries are the sternum anteriorly, the vertebral column posteriorly, and the medial parietal pleural surfaces of the right and left lungs laterally. The mediastinum contains four compartments: the superior, anterior, middle, and posterior (Fig. 60-2). The superior mediastinum lies in the thoracic space cephalad to a plane joining the fourth
thoracic vertebra and the sternomanubrial junction. The anterior mediastinum is bounded superiorly by the superior mediastinum, inferiorly by the diaphragm, anteriorly by the sternum, and posteriorly by the pericardium. The middle mediastinum is bounded by the anterior and posterior limits of the pericardium. The posterior mediastinum is bounded superiorly by the superior mediastinum, inferiorly by the diaphragm, anteriorly by the pericardium, and posteriorly by the vertebral column.
thoracic vertebra and the sternomanubrial junction. The anterior mediastinum is bounded superiorly by the superior mediastinum, inferiorly by the diaphragm, anteriorly by the sternum, and posteriorly by the pericardium. The middle mediastinum is bounded by the anterior and posterior limits of the pericardium. The posterior mediastinum is bounded superiorly by the superior mediastinum, inferiorly by the diaphragm, anteriorly by the pericardium, and posteriorly by the vertebral column.
![]() FIGURE 60-2. Anatomic division of the mediastinum into superior, anterior, middle, and posterior compartments. |
The right and left pleural spaces are separate entities that extend from the lateral aspects of the mediastinum as they envelope each lung. The parietal pleura lines the thoracic wall, whereas the visceral pleura adheres intimately to the pulmonary surface. A thin layer of mesothelial cells separates the two surfaces (10). In some areas, the parietal pleura may fold onto itself until the lung intercedes during inspiration. These areas are found inferiorly along the edges of the diaphragm, in the costodiaphragmatic sinus, and in a small cleft behind the sternum known as the costomediastinal sinus (10). The blood supply of the parietal pleura is derived from the intercostal, internal mammary, superior phrenic, and anterior mediastinal arteries. Corresponding veins drain the parietal pleura into the systemic veins. The visceral pleura is supplied by bronchial and pulmonary artery radicals; however, venous drainage is only to the pulmonary circulation (10). The parietal pleura receives sensory innervation from the intercostal and phrenic nerves. This results in relatively precise sensory localization. The visceral pleura receives vagal and sympathetic innervation and has far less precise sensory localization (10).
A dynamic relationship between the systemic and pulmonary vasculature and the lymphatic circulation maintains a relatively constant amount of pleural fluid, which is evenly distributed within the pleural space (11,12). Most pleural fluid is formed from the systemic circulation and travels along pressure gradients in the pleural space until it is primarily resorbed by parietal pleural lymphatics. Elevation of systemic venous pressure or lymphatic pressure results in excessive accumulation of pleural fluid either by increased production (venous congestion) or decreased absorption (lymphatic congestion). Normally, therefore, there is little interchange between pleural and pulmonary fluids. Pulmonary edema can disturb this balance by presenting a greater amount of fluid to the visceral pleura from the pulmonary parenchyma itself (13).
PHYSIOLOGY
The anatomy of the pleural space plays a significant role in respiratory physiology. During quiet respiration, the intrapleural pressure is -8 to -9 mm Hg during inspiration and -3 to -6 mm Hg during expiration. A positive gradient exists between the intrabronchial pressure and intrapleural pressure throughout the respiratory cycle, thus holding the pleural surfaces together (10). During inspiration, the lung is expanded by the negative pressure generated by the movement of the diaphragm and thoracic wall. In normal breathing, the diaphragmatic excursion is only about 2 cm. If the diaphragm is paralyzed, as seen in eventration of the diaphragm, it moves paradoxically upward during inspiration in response to the fall in intrathoracic pressure, interfering with ventilation of the ipsilateral lung (Fig. 60-3). The major accessory muscles of inspiration include the scalene and sternocleidomastoid muscles. These are usually quiescent during normal breathing, but may be quite active during exercise or respiratory insufficiency (14). Expiration is usually a passive event, but may become active during exercise and hyperventilation. The abdominal wall muscles are the most important muscles in active expiration, but are aided by intercostal muscles (14).
Various factors influence the efficiency of ventilation. First, the chest wall is quite elastic. There is an ongoing tension between the lung, which naturally tends to recoil inward, and the thoracic wall, which tends to recoil outward. This tension helps to keep the lung expanded. At rest, the upper lung is relatively more expanded than the lower lung because the intrathoracic pressure is less negative at the base than at the apex due to gravity. During inspiration, however, the lower lung expands more, relative to the upper lung, demonstrating a greater compliance (15). Gravity is not the lone determinant of this vertical gradient. The shapes of the intrathoracic organs, the chest wall orientation, and the tendency of the structures to resist displacement or deformation contribute to this gradient (16,17).
In fact, the two lungs can function almost as separate structures with different mechanical properties (16). The position of the patient may also affect the distribution of ventilation within the lung. For example, experimental studies have shown an improvement in oxygenation in animals placed in the prone versus the supine position because small airway patency is better maintained in the prone position (18).
In fact, the two lungs can function almost as separate structures with different mechanical properties (16). The position of the patient may also affect the distribution of ventilation within the lung. For example, experimental studies have shown an improvement in oxygenation in animals placed in the prone versus the supine position because small airway patency is better maintained in the prone position (18).
The previous section describes the respiratory status of the child and the adult, but there are special considerations in the infant. Anatomic and physiologic dynamics are not fully developed in the neonate. The overly compliant chest wall is easily distorted during respiratory efforts. This is especially true during sleep because intercostal muscle activity is reduced, resulting in decreased lung dynamic compliance. Neonatal lung tissue is more viscous, peripheral airways offer more resistance, and mismatches of ventilation and perfusion are more marked than in the older child and adult. The upper airway is quite pliable and can close at pressures of 4 mm Hg. Neck extension can enhance airway patency, whereas flexion can hinder it. In addition, the functional residual capacity (FRC) in the neonate comprises a relatively higher proportion of lung capacity due to a decreased expiratory phase. This helps in the absorption of intrapulmonary fluid and the maintenance of a more uniform airway expansion, but leaves the infant vulnerable to atelectasis (19).
OPERATIVE PRINCIPLES
Anesthetic considerations are extremely important for infants and children undergoing thoracic surgery. The normal cardiopulmonary physiology in these young patients may compromise their ability to respond to the changes induced by general anesthesia. Infants and children have a relatively small FRC compared with tidal volume. In newborns, the FRC actually overlaps the closing volume of the lung (20). Thus, newborns are especially prone to alveolar collapse during open chest anesthesia. The use of positive end-expiratory pressure (PEEP) may prevent atelectasis and eliminate clinically significant hypoxia. The
infant’s myocardium is relatively less sensitive to the positive inotropic effects of isoproterenol and dopamine, and relatively more sensitive to the negative inotropic effects of propranolol (21). The infant’s myocardium is also less compliant than that of the older child. Because of this difference in physiology, the cardiac output in infants is augmented principally by increasing heart rate, at a relatively fixed stroke volume. As the heart rate increases, however, there is less time for ventricular filling. A point is reached at which the infant can no longer increase cardiac output by this means and shock ensues. Shock may develop rapidly in infants, and is often heralded only by a progressive increase in heart rate, rather than a gradual decline in blood pressure. If the response to inotropic support is inadequate, vigorous volume resuscitation is necessary to support the peripheral circulation.
infant’s myocardium is relatively less sensitive to the positive inotropic effects of isoproterenol and dopamine, and relatively more sensitive to the negative inotropic effects of propranolol (21). The infant’s myocardium is also less compliant than that of the older child. Because of this difference in physiology, the cardiac output in infants is augmented principally by increasing heart rate, at a relatively fixed stroke volume. As the heart rate increases, however, there is less time for ventricular filling. A point is reached at which the infant can no longer increase cardiac output by this means and shock ensues. Shock may develop rapidly in infants, and is often heralded only by a progressive increase in heart rate, rather than a gradual decline in blood pressure. If the response to inotropic support is inadequate, vigorous volume resuscitation is necessary to support the peripheral circulation.
Pulmonary physiology in the lateral decubitus position has been thoroughly studied in adult patients, but poorly evaluated in children. Gravity increases the pulmonary blood flow to the dependent lung, as compared with the nondependent lung. Conversely, ventilation is superior in the nondependent lung because of elevation of the dependent diaphragm and gravitational shifts of the mediastinum (22) (Fig. 60-4). These changes in ventilation may be even more significant in small children because the mediastinum is more mobile and the abdominal organs are relatively larger. An anesthetized, paralyzed child in the lateral decubitus position, therefore, may experience significant ventilation–perfusion mismatch. The reduction of regional ventilation in the child’s dependent lung may precipitate alveolar collapse and systemic hypoxia. This ventilation–perfusion mismatch may be further exacerbated by one-lung ventilation techniques, such as those used for many thoracoscopic procedures. In this instance, all the blood flow to the nondependent and nonventilated lung becomes shunt flow, creating an obligatory right-to-left shunt. Increasing the FIO2 and respiratory rate may be enough to compensate for this shunt fraction and allow the procedure to be completed, but some infants and children become significantly hypoxic under these conditions and cannot tolerate one-lung ventilation in this position. The use of positive-pressure ventilation with PEEP prevents alveolar collapse and maximizes ventilation of the dependent lung.
Maintaining the fluid and glucose balance in children undergoing thoracic surgery is critical. Fluid overload in neonates may open a previously closed ductus arteriosus, creating a significant shunt. Likewise, excessive administration of crystalloid during an operative procedure may precipitate interstitial pulmonary edema, further aggravating the potential for systemic hypoxia. Infants undergoing thoracic surgery should generally receive no more than 75% of their calculated maintenance fluid volume in the first 24 to 48 hours postoperatively, unless there are ongoing fluid losses. Reductions in fluid intake are usually accompanied by reductions in glucose intake. Thus, careful glucose monitoring is important during this interval.
Intraoperative and perioperative monitoring of cardiac and respiratory function is essential in infants and children because their clinical condition may change rapidly. In the past, the size of these patients often made sophisticated monitoring of cardiopulmonary function difficult or impossible, but modern equipment has largely overcome these difficulties. As a minimum, all children undergoing thoracic surgery should have continuous monitoring of heart rate, blood pressure, electrocardiogram, oxygen saturation, and temperature. Blood pressure monitoring is facilitated by Doppler equipment, such as the Dinamap. This instrument can repeatedly measure systolic, diastolic, and mean blood pressure at preset time intervals in patients of all sizes. Automated blood pressure readings correlate closely with intraarterial readings, if appropriate size cuffs are used (23).
Oxygen saturation can be continuously measured with transcutaneous sensors. This equipment can be used on patients of all ages, and the readings are not adversely affected by the percentage of fetal hemoglobin in the circulation. Because of the shape of the oxyhemoglobin dissociation curve, oxygen saturation provides a better method for detecting levels of hypoxia than for detecting hyperoxia. This is not of practical importance, except in small premature infants in whom persistent excessive oxygenation can affect the retinal circulation. When oxygen saturations
are maintained at or below 95%, the PaO2 is not likely to exceed 100 mm Hg (24) (Fig. 60-5). Maintaining saturation levels between 85% and 95% assures a safe range of partial pressure of oxygen for premature infants. The ability to continuously monitor oxygen saturation has provided a sensitive method for detecting mechanical anesthetic complications, such as mainstem intubation or obstruction of the endotracheal tube. Using these devices in the postoperative period has greatly reduced the need for arterial blood gas sampling. Two oxygen saturation sensors can be used to detect right-to-left shunting through the ductus arteriosus. One sensor is placed proximal (right arm) and one is placed distal (legs) to the ductus arteriosus. A difference of greater than 5% saturation between the two sensors indicates significant shunting. Advances in technology have created accurate and practical end-tidal CO2 monitors. These devices are connected to the endotracheal tube and sample expired gas concentrations. They provide an accurate correlation with arterial PCO2, except in infants who weigh less than 4 kg. In these small patients, sampling errors of the small tidal volume may lead to inaccurate readings (25).
are maintained at or below 95%, the PaO2 is not likely to exceed 100 mm Hg (24) (Fig. 60-5). Maintaining saturation levels between 85% and 95% assures a safe range of partial pressure of oxygen for premature infants. The ability to continuously monitor oxygen saturation has provided a sensitive method for detecting mechanical anesthetic complications, such as mainstem intubation or obstruction of the endotracheal tube. Using these devices in the postoperative period has greatly reduced the need for arterial blood gas sampling. Two oxygen saturation sensors can be used to detect right-to-left shunting through the ductus arteriosus. One sensor is placed proximal (right arm) and one is placed distal (legs) to the ductus arteriosus. A difference of greater than 5% saturation between the two sensors indicates significant shunting. Advances in technology have created accurate and practical end-tidal CO2 monitors. These devices are connected to the endotracheal tube and sample expired gas concentrations. They provide an accurate correlation with arterial PCO2, except in infants who weigh less than 4 kg. In these small patients, sampling errors of the small tidal volume may lead to inaccurate readings (25).
Catheters are available for measuring pulmonary capillary wedge pressure and performing calorimetric cardiac output in pediatric patients. In infants, these catheters (4F) may need to be inserted by cutdown techniques, but in older children they may be placed percutaneously. Percutaneous arterial catheters (22 gauge and 24 gauge) can be used in infants and children. Usually, these are placed in the radial artery, although the femoral artery may be used in small infants for short-term monitoring.
Certain relatively common thoracic surgical procedures in children require special anesthetic considerations. Airway endoscopy is frequently performed in children to evaluate the etiology of stridor or to remove airway foreign bodies. Most of these procedures are performed under general anesthesia, using a rigid ventilating bronchoscope. Insertion of this instrument in small children, even under general anesthesia, may precipitate laryngospasm. Applying topical anesthesia (lidocaine 4%) to the vocal cords before introducing the bronchoscope reduces the hazard of laryngospasm and eliminates much of the discomfort of the procedure. Positive-pressure ventilation through the bronchoscope is contraindicated in many of the bronchoscopic procedures performed in children. For example, positive pressure used in patients with airway foreign bodies may impact the foreign body onto the carina or into a distal bronchus. In addition, patients with pulmonary air trapping, such as children with lobar emphysema who are undergoing bronchoscopy for selective airway aspiration, may experience sudden respiratory collapse with positive-pressure ventilation because of the rapid expansion of the involved lobe or development of a pneumothorax. Rah et al. documented the inadequacies of ventilation with the smallest bronchoscopes (2.5 and 3 mm) when the rod lens telescope is in place (26). The lumen available for ventilation under these circumstances is very small, and exhalation of gas from the lung is impeded. When using these bronchoscopes, the surgeon is advised to remove the telescope intermittently, allowing periods of ventilation to avoid severe hypercarbia or pneumothorax.
Pain relief in children undergoing thoracic surgery is important to minimize respiratory complications. Intrapleural catheters inserted after the operation is completed provide excellent postoperative analgesia with intermittent or continuous infusion of bupivacaine (27,28). Regional anesthetic techniques, including local infiltration of the surgical wound and intercostal nerve blocks, may also help. Older children can be safely treated with patient-controlled analgesia pumps that allow intravenous infusion of measured doses of analgesics (29). Epidural catheters may be employed in some settings for perioperative analgesia (30).
The standard operative approach for most pediatric thoracic procedures has traditionally been the posterolateral thoracotomy. This incision divides a portion of the latissimus dorsi muscle and the serratus anterior muscle beneath it. For many years, a rib resection was advocated for the retropleural dissection used in correcting esophageal atresia with tracheoesophageal fistula.
Currently, however, an intercostal approach is preferred in an effort to avoid growth disturbances of the chest wall in these patients with considerable growth ahead of them. Chetcuti et al. reviewed a series of 302 patients who had survived esophageal atresia repair for four decades (31). Of patients who underwent a rib resection thoracotomy, 38% developed anterior chest wall asymmetry, scoliosis, or both. However, 25% of patients who underwent an intercostal thoracotomy without rib resection developed similar skeletal abnormalities. These deformities appeared to be progressive because their incidence increased with longer follow-up. Their incidence also increased significantly when more than one thoracotomy was necessary. The authors believed that the major factor that produced anterior chest wall asymmetry was partial denervation of the serratus anterior with subsequent atrophy of this muscle, rather than the rib resection itself. The development of scoliosis was more common in patients who had congenital vertebral anomalies, but was believed to be secondary to interference with intercostal muscle function in patients with normal spines. Jaureguizar et al. followed 89 patients after correction of esophageal atresia (32). Of these patients, 24% developed shoulder elevation on the side of the thoracotomy and 20% were found to have chest wall deformities, including asymmetric breast growth in female patients. Denervation of the serratus anterior muscle was also believed to be responsible for these abnormalities. Goodman et al. reported atrophy of both the serratus anterior and latissimus dorsi muscles in computed tomography (CT) studies of patients following a posterolateral thoracotomy (33). Because of these and similar studies, many pediatric surgeons advocate the of use muscle-sparing thoracotomy incisions (34,35,36). The incision for these thoracotomies extends posteriorly from the mid axillary line to avoid interference with breast development. The latissimus dorsi muscle can be mobilized and retracted either posteriorly or anteriorly, depending on the exposure required. With anterior retraction of the latissimus, the chest can be entered through the triangle of auscultation without dividing or mobilizing the serratus muscle. With posterior retraction, the inferior border of the serratus is mobilized and retracted anteriorly to allow entry through the fourth intercostal space (Fig. 60-6). These incisions lend themselves nicely to most intrathoracic procedures in infants and children. Although there are no long-term follow-up studies of chest wall development in children following these procedures, it is believed that avoiding division and denervation of these major muscles minimizes the chest wall and spinal deformities that occur following standard thoracotomy procedures. Ponn et al. evaluated 62 adult patients undergoing muscle-sparing thoracotomy 6 months after surgery. In these patients, forced vital capacity was significantly better preserved than in patients undergoing standard muscle-splitting thoracotomies (37).
Currently, however, an intercostal approach is preferred in an effort to avoid growth disturbances of the chest wall in these patients with considerable growth ahead of them. Chetcuti et al. reviewed a series of 302 patients who had survived esophageal atresia repair for four decades (31). Of patients who underwent a rib resection thoracotomy, 38% developed anterior chest wall asymmetry, scoliosis, or both. However, 25% of patients who underwent an intercostal thoracotomy without rib resection developed similar skeletal abnormalities. These deformities appeared to be progressive because their incidence increased with longer follow-up. Their incidence also increased significantly when more than one thoracotomy was necessary. The authors believed that the major factor that produced anterior chest wall asymmetry was partial denervation of the serratus anterior with subsequent atrophy of this muscle, rather than the rib resection itself. The development of scoliosis was more common in patients who had congenital vertebral anomalies, but was believed to be secondary to interference with intercostal muscle function in patients with normal spines. Jaureguizar et al. followed 89 patients after correction of esophageal atresia (32). Of these patients, 24% developed shoulder elevation on the side of the thoracotomy and 20% were found to have chest wall deformities, including asymmetric breast growth in female patients. Denervation of the serratus anterior muscle was also believed to be responsible for these abnormalities. Goodman et al. reported atrophy of both the serratus anterior and latissimus dorsi muscles in computed tomography (CT) studies of patients following a posterolateral thoracotomy (33). Because of these and similar studies, many pediatric surgeons advocate the of use muscle-sparing thoracotomy incisions (34,35,36). The incision for these thoracotomies extends posteriorly from the mid axillary line to avoid interference with breast development. The latissimus dorsi muscle can be mobilized and retracted either posteriorly or anteriorly, depending on the exposure required. With anterior retraction of the latissimus, the chest can be entered through the triangle of auscultation without dividing or mobilizing the serratus muscle. With posterior retraction, the inferior border of the serratus is mobilized and retracted anteriorly to allow entry through the fourth intercostal space (Fig. 60-6). These incisions lend themselves nicely to most intrathoracic procedures in infants and children. Although there are no long-term follow-up studies of chest wall development in children following these procedures, it is believed that avoiding division and denervation of these major muscles minimizes the chest wall and spinal deformities that occur following standard thoracotomy procedures. Ponn et al. evaluated 62 adult patients undergoing muscle-sparing thoracotomy 6 months after surgery. In these patients, forced vital capacity was significantly better preserved than in patients undergoing standard muscle-splitting thoracotomies (37).
THORACOSCOPY
The desire for a minimally invasive and minimally deforming thoracotomy has naturally led pediatric surgeons to explore thoracoscopy for many intrathoracic procedures. In thoracoscopy, the pleural cavity is accessed by instruments placed through trocars that are inserted through the intercostal space, without spreading the ribs. The intercostal muscles are not divided, and the muscles of the chest wall are left undisturbed. Initially proposed in 1976 as a technique for obtaining pulmonary biopsies in immunocompromised children, thoracoscopy has now been applied to a diverse range of thoracic disorders in children (38). With the development of sophisticated dissecting and retracting instruments, many therapeutic procedures can now be performed in the thoracic cavity with these instruments (Fig. 60-7). The success of thoracoscopy depends on the ability to establish a sufficient pneumothorax that allows visualization of the area of pathology. Patients with extensive pleural symphysis are thus not good candidates for thoracoscopic procedures. Careful preoperative imaging is essential for planning appropriate patient position and trocar location. Most thoracoscopy procedures are best performed in a full lateral decubitus position, although procedures involving lesions in the anterior or posterior mediastinum may be more easily accomplished by placing the patient in a 45-degree lateral position or an exaggerated lateral position, respectively.
Procedures involving retraction of the lung or extensive dissection within the mediastinum are best performed under general anesthesia, using either tracheal or selective mainstem ventilation. Selective mainstem intubation has the advantage of allowing complete collapse of the ipsilateral lung, thus avoiding the need for retraction. Positive-pressure ventilation in patients with tracheal ventilation may interfere with maintenance of an ipsilateral pneumothorax, reducing the visibility in the operated chest. Infusing carbon dioxide gas under low pressure (4 to 6 mm Hg) and low flow (100 mL per min) may alleviate this problem. Simple procedures, such as pleurodesis or pulmonary biopsy, can be performed under regional anesthesia, using an intercostal block with additional intravenous sedation as needed (39). After insertion of the initial trocar and telescope, the entire hemithorax is evaluated for areas of unsuspected pathology. Planning is then completed for insertion of the trocars for dissecting and retracting instruments. The lesion is then dissected entirely from its surrounding structures or biopsied. Hemostasis can be achieved either with the insulated electrocautery, metallic clips, endoscopic stapling, or ultrasonic dissecting shears. A chest tube is inserted through one of the trocar tracts, and the remaining tracts are closed in layers. In most patients, a small catheter is left through one of the trocar tracts for instillation of intrapleural analgesia.
Thoracoscopy has become widely accepted for use in pediatric patients for a variety of indications. Currently, one of the most frequent indications is for pleural debridement in children with fibrinopurulent empyema. This technique is easy to learn and has been virtually 100% successful in quickly clearing the pleural infection and facilitating discharge of these patients (40,41). Thoracoscopy is also commonly used for biopsy or excision of mediastinal lesions, for pulmonary biopsy in patients with diffuse or localized interstitial infiltrates, and for pleurodesis in treatment of spontaneous pneumothorax. Thoracoscopic biopsy or excision of mediastinal lesions has been successful in more than 95% of the children reported, and there have been no significant complications when using the technique for this purpose (42). Preoperative imaging is especially important for these patients to ensure they are appropriately positioned to allow access to the area of pathology. Cross-sectional scans are helpful to define the relationship of the lesion to vital structures in the vicinity. For simple biopsy of mediastinal lesions, two trocars are usually sufficient; one for the telescope and one for the biopsy forceps. Additional trocars may be necessary for retraction of the lung or if complete excision of the lesion is anticipated. Several authors have described complete excision of bronchogenic cysts and neurogenic tumors of the mediastinum in children using thoracoscopy (42,43,44,45). The cysts may be aspirated under direct vision and removed through one of the trocar tracts in an endosac. Mediastinal tumors should be placed in an Endosac and may be removed through a slightly expanded trocar tract. The use of thoracoscopy to biopsy mediastinal lymphadenopathy has proven particularly helpful in pediatric patients.
Patients with bulky lesions with some element of tracheal compression may be biopsied under regional anesthesia, thus reducing the anesthetic hazard of the procedure.
Patients with bulky lesions with some element of tracheal compression may be biopsied under regional anesthesia, thus reducing the anesthetic hazard of the procedure.
The use of thoracoscopy to provide accurate tissue diagnosis in children with diffuse or localized pulmonary infiltrates has been successful in nearly 100% of the patients reported (45). These biopsies may be rapidly obtained using the endoscopic stapling device to minimize postoperative air leaks or bleeding. This device must be passed through a l2-mm trocar and must be able to be passed at least 5 cm into the chest to fully open the anvil. It is therefore not applicable for use in very small patients, usually those younger than 3 or 4 years. More recently, the ultrasonic scalpel has been used for this purpose with success, and these instruments may be passed through a 5-mm trocar (46).
Most complications of thoracoscopy in children have occurred in patients undergoing lung biopsy who are immunocompromised and on mechanical ventilation (45). The problems in obtaining hemostasis and pneumostasis after these procedures have occasionally led to serious complications, including bleeding and bronchopleural fistulas. Many of these children should be biopsied by open techniques that provide better parenchymal control.
Thoracoscopic treatment of pneumothorax can be accomplished by various methods, including talc pleurodesis, mechanical pleural abrasion, and pleurectomy. Talc pleurodesis and pleurectomy have been successful in virtually 100% of the patients in whom they have been used (47). Mechanical pleural abrasion is successful in 90% to 95% of patients (48). Talc pleurodesis is simple to perform and can be completed under regional anesthesia, whereas pleurectomy and pleural abrasion cause more discomfort and require general anesthesia (39). With any of these techniques, blebs or cysts can be excised using either the endoscopic stapler or ultrasonic scalpel. Thoracoscopy can be used to treat chylothorax, either by occluding the thoracic duct with metallic clips or sutures or by spraying the mediastinum with fibrin glue (49).
As pediatric surgeons have become more comfortable with the technique of thoracoscopy and more sophisticated instruments have been developed, this technique is being applied to many more complicated intrathoracic procedures in children. Several authors have reported formal anatomic pulmonary lobectomy, even in very small children (50). Thoracoscopy is now frequently used for interruption of the ductus arteriosis in small infants and several authors have described using the technique for division of vascular rings (51,52). More recently, pediatric surgeons have begun to repair infants with esophageal atresia and tracheoesophageal fistula with thoracoscopic techniques with excellent results (53,54).
The introduction of robotic equipment for minimally invasive surgery has stimulated pediatric surgeons to explore its usefulness in pediatric thoracoscopy. This equipment, although somewhat complex to set up, has the important advantage of 6 or 7 degrees of movement of the endoscopic instruments, allowing for considerable flexibility while working in small spaces (55). Clinical reports have appeared of resection of mediastinal tumors with robotic equipment and patent ductus arteriosus, and vascular rings have been successfully divided in children as small as 2 to 3 kg (52,56,57). Repair of esophageal atresia has been simulated in experimental animals using robotic technology (58,59). It is anticipated that the use of this equipment will see wider application in pediatric surgical practice as the instruments are refined and scaled down for pediatric use.
PNEUMOTHORAX
A pneumothorax is an accumulation of air within the pleural space. It may occur spontaneously or as the result of trauma, surgery, or a therapeutic intervention. If the air accumulates under pressure, a tension pneumothorax ensues. A pneumothorax decreases pulmonary volume, compliance, and diffusing capacity. If the pneumothorax is greater than 50% of chest volume, hypoxia may result secondary to ventilation–perfusion mismatch. A normal lung can often compensate this for. Children with underlying chronic pulmonary disease may develop significant relatively smaller pneumothoraces secondary to diminished elastic recoil of their lungs, but the symptomatic consequences may be more significant because of their small margin of pulmonary reserve (60,61,62). Spontaneous pneumothorax may occur in children with no known underlying disease or may result from or, in fact, reveal an underlying condition, such as a congenital bleb, pneumonia with pneumatocele or abscess, tuberculosis, or cystic adenomatoid malformation. Traumatic pneumothorax may result from a tear in the pleura, esophagus, trachea, or bronchi. Iatrogenic causes include mechanical ventilation, thoracentesis or central venous catheter insertion, bronchoscopy, or cardiopulmonary resuscitation (60,63,64,65).
The most common presenting symptoms of pneumothorax are ipsilateral chest pain and dyspnea (60,64). Severe dyspnea should alert the surgeon to the presence of a tension pneumothorax. Physical examination may reveal diminished breath sounds on one side of the chest or a shift of the trachea from the suprasternal notch. A pneumothorax is usually detectable on a chest radiograph and is enhanced if the radiograph is taken at end expiration. It is common practice for the size of a pneumothorax to be described as a proportion of the chest field on an upright radiograph. The actual volume loss of the lung is greater than such a description because pulmonary volume is lost in three dimensions. The following formula is used to more accurately estimate this loss:

![]() FIGURE 60-8. A nomogram for predicting the percentage of involvement with a pneumothorax in larger children and adolescents. |
A number of factors determine the proper management of a pneumothorax. These include the initial size, symptomatology, ongoing expansion, presence of tension, and any contributing underlying condition. A spontaneous unilateral pneumothorax that is asymptomatic and less than 15% to 20% of the chest volume can usually be followed by observation alone (61,65). Pleural air reabsorbs at a rate of 1,25% per day, but this can be hastened by breathing supplemental oxygen (61,67). Classically, such a pneumothorax occurs in an ectomorphic, adolescent male. If there is no known underlying pathology, the chances are that such an episode represents the rupture of a subpleural bleb or cyst, the manifestation of a familial tendency, or perhaps even the presence of undetected tuberculosis (68). Pneumothorax recurs with a frequency of 50% after the first episode, 62% after the second, and 83% after the third (61,69).
Symptomatic or large pneumothoraces usually require intervention. Options for treatment of a small pneumothorax include thoracentesis, placement of a unidirectional (Heimlich-type) valve, or placement of a small pigtail catheter (70,71,72). However, if air is continuously aspirated or if the pneumothorax recurs, a standard chest tube should be inserted. A tension pneumothorax poses a surgical emergency and a chest tube should be placed immediately. If a chest tube is not immediately available or if the patient’s condition deteriorates during preparation for placement, a large-bore (14-gauge) needle should be placed in the second intercostal space anteriorly to relieve the tension. If the pneumothorax recurs after tube thoracostomy, or if the air leak persists, further intervention is necessary. The choice of intervention is determined by the cause of the condition. In a posttraumatic pneumothorax, large or persistent air leaks may indicate damage to the airway or the esophagus. Appropriate diagnostic studies using esophagograms, bronchoscopy, esophagoscopy, thoracoscopy, or thoracotomy should be undertaken and direct repair of the injury, if present, performed. If the air leak is due to lung parenchymal injury, chest tube drainage is usually adequate. In a nontraumatic pneumothorax, the persistence of an air leak may accompany a chronic underlying condition, such as cystic fibrosis, bronchopulmonary dysplasia, lung cysts, or blebs. Treatment of these patients usually requires resection of the local pathology or pleurodesis.
Pleurodesis can be undertaken by instilling chemical agents into the pleural space through a chest tube, by thoracoscopy, or by thoracotomy. In the past, agents such as silver nitrate, quinacrine, iodized oil, and hypertonic glucose have been used, but currently, talc or tetracycline derivatives, such as doxycycline or fibrin sealant, are more commonly used (47,73,74,75,76,77). Talc is less painful and more uniformly successful than doxycycline. Treatment with talc has been shown to be particularly effective in treating pneumothoraces in children with cystic fibrosis (47). A more recent study in adults does suggest that talc may have systemic distribution and may rarely produce adult respiratory distress syndrome (78). Autologous blood patching may be useful in preventing pneumothorax after lung biopsy (79).
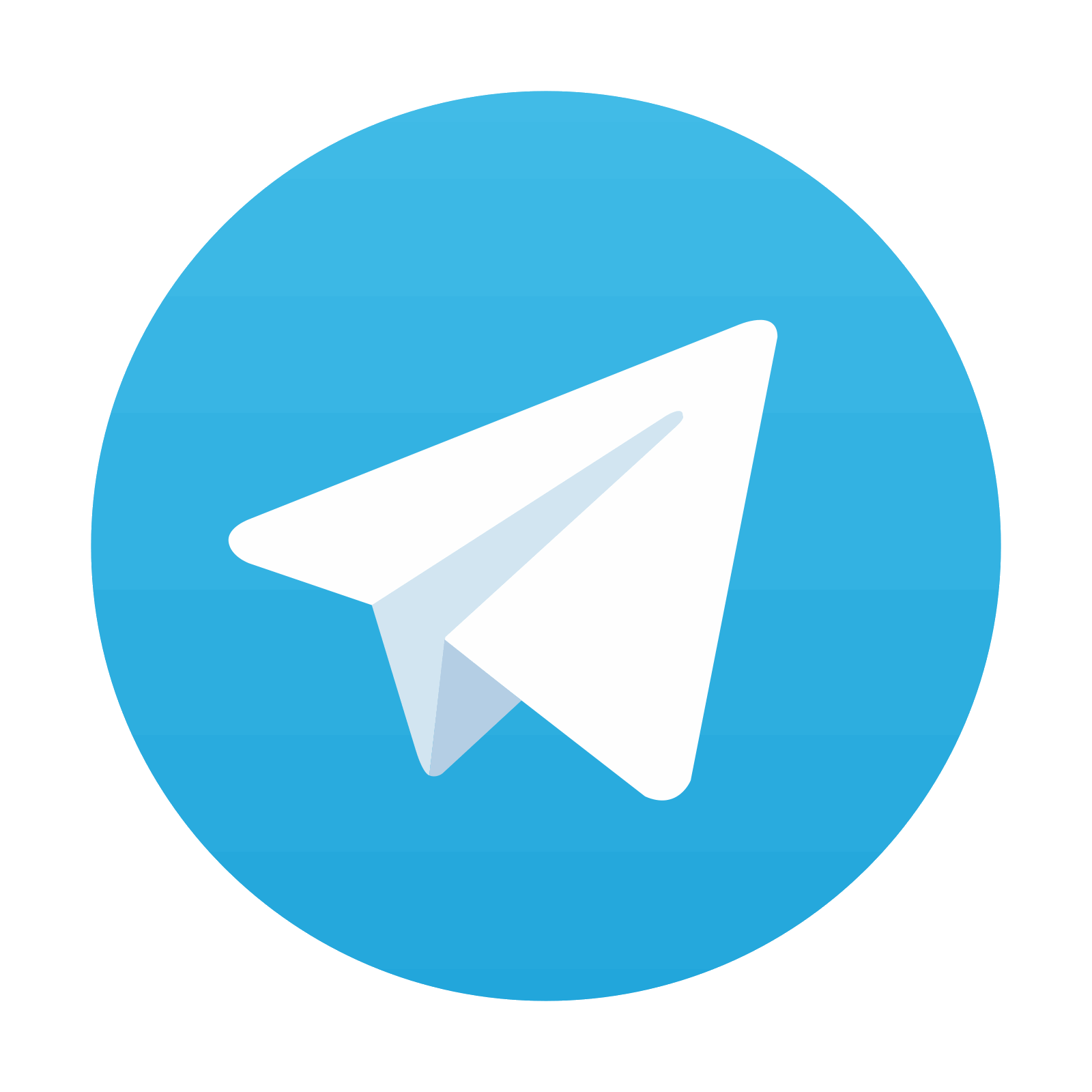
Stay updated, free articles. Join our Telegram channel
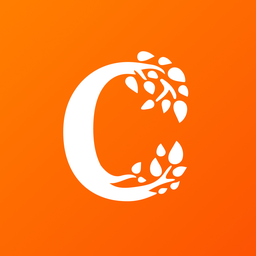
Full access? Get Clinical Tree
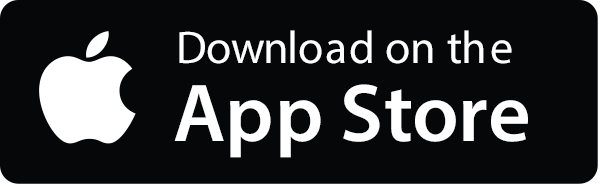
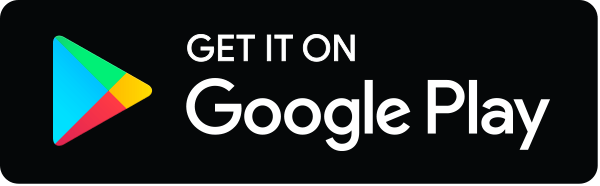