i. Increased pulmonary blood flow, to about 10 times fetal levels. In utero pulmonary blood flow is low as a function of the vasoconstricted state of pulmonary blood vessels. This occurs in part due to the exposure of the pulmonary vascular bed to higher alveolar concentrations of oxygen as compared to the relatively hypoxic intrauterine environment. Other metabolically active substances, such as metabolites of prostaglandin, bradykinins, or histamine, may play some role through inducing pulmonary vasodilation.
ii. Alteration in flow through fetal channels such as the ductus arteriosus and foramen ovale that may last for many days. The major change in flow through fetal channels is either a direct result of increased flow to the lungs or higher arterial oxygen tension. The augmentation of pulmonary venous return leads to an increase in left atrial pressure, which causes displacement of the flap of the foramen ovale over the rims of the fossa, thus abolishing any right-to-left atrial flow. There is oftentimes some residual left-to-right transatrial flow for a period of time as the circulation readjusts. The pattern of flow through the ductus arteriosus is influenced by both systemic and pulmonary vascular resistance (PVR). As lung compliance improves, PVR decreases leading to a reversal in the directionality of the shunt from right to left to bidirectional and eventually left to right. The increase in systemic vascular resistance (SVR), upon removal of the compliant placenta from the systemic circuit and as a result of increased systemic arterial oxygen tension, further exaggerates this change. Recent normative data from term human cohorts suggest that the transductal shunt is left to right by 24 hours, after which a persistent right-to-left shunt should be considered pathologic (1). In premature infants, the administration of surfactant can alter transductal flow significantly through reduced PVR. Finally, the processes leading to ductal closure differ between term and premature infants. The architecture of the immature arterial duct differs such that ductal tone is less responsive to oxygen, thus delaying closure and potentially contributing to excessive flow to the lungs and compromised systemic flow. There is evidence of functional closure by 6 hours in some immature patients, although this is rare (2).
iii. Increased left ventricular output (LVO) and right ventricular output (RVO) are necessary to meet the metabolic needs of immature neonate with insufficient thermoregulatory mechanisms and increased work of breathing. The increase in RVO relates to improved systemic blood flow and lower right ventricular (RV) afterload secondary to pulmonary vasodilation. The transition from right-to-left ventricular dominance occurs over hours and is secondary to increased left atrial preload and left ventricular (LV) afterload. In total, there is a threefold increase in LVO, which is necessary to meet the increased demands of the body. The enhanced ability of the left ventricle to increase its output is related, in part, to elimination of constraint by the pressure-loaded right ventricle.
the systemic blood flow is normal. Elevation in heart rate beyond 160 beats per minute or a sustained elevation from baseline may indicate hypovolemia. However, many noncardiovascular factors can also influence heart rate. Elevated heart rate can be caused by pain, hyperthermia, and commonly used medications such as caffeine and atropine. Sinus bradycardia occurs commonly in sleep, and the neonate should respond with increased heart rate when awakened. Other factors that should be considered if the heart rate remains low include hypothermia, hypothyroidism, electrolyte imbalance, and some medications such as β antagonists (e.g., propranolol).
TABLE 29.1 Clinical Indicators of Cardiovascular Health | ||||||||||||||||||||||||||||||||||||
---|---|---|---|---|---|---|---|---|---|---|---|---|---|---|---|---|---|---|---|---|---|---|---|---|---|---|---|---|---|---|---|---|---|---|---|---|
|
(<70%) is associated with negative outcomes in children, but neonatal studies are lacking (12). Poorly perfused organs in situations of low systemic blood flow extract as much oxygen as possible to optimize aerobic metabolism; hence svO2 will be low. Caution should be used interpreting svO2 in disorders such as necrotizing enterocolitis (NEC) as ischemic tissue does not extract oxygen and therefore high svO2 may be misleading. In neonates, catheter positioning is difficult, and acquiring a mixed svO2, for which the optimal catheter position is the main pulmonary artery is not typically possible. Central venous saturation, which is optimally acquired from the right atrium at the junction of the superior vena cava (SVC) or inferior vena cava (IVC), can be measured and changes in parallel with mixed svO2. The absolute values of mixed and central svO2 are not interchangeable, and central svO2 is affected by catheter position. Due to differential blood flow to vital organs in conditions of shock, a catheter placed in the SVC will overestimate mixed svO2, while one in the IVC will underestimate it. The presence of a high-volume peripheral shunt decreases systemic organ perfusion and increases pulmonary blood flow and hence leads to overestimation of svO2. If catheter position is appropriate, changes in central svO2 over time can be helpful in assessment of neonates with low CO.
to bioimpedance, which aims to detect changes in the amplitude of an applied electrical current, bioreactance estimates changes in the frequency of the current (the relative phase shift) between the input and output signals, which is induced by blood ejected into the aorta from the left ventricle. Stroke volume is estimated using the measured peak rate of change of the phase shift, ventricular ejection time, and a constant of proportionality that accounts for patient age, gender, and body size. Studies have reported variable reliability of NICOM-measured CO. NICOM was compared with invasive measurements of CO using an aortic root catheter in anaesthetized beagles and demonstrated a bias of 63 ± 38 mL/min, a percent bias of 6.1%, and high responsiveness to pharmacologically induced changes in CO (22). In contrast, NICOM demonstrated poor reliability and responsiveness compared with pulmonary artery thermodilution-measured CO in critically ill adults being treated with volume expansion (mean bias 0.9 L/min/m2 and limits of agreement -2.2 to 4.1) (23). In a heterogeneous group of term and moderately preterm neonates, NICOM estimates of CO were strongly correlated with TTE (r = 0.95) but NICOM systematically underestimated CO by 31 ± 8% (24). In a cohort of extremely preterm infants undergoing PDA ligation, NICOM similarly underestimated CO relative to echocardiography (mean bias 39%, limits of agreement 8% to 69%), with increasing bias over time (25). Collectively, these studies suggest that NICOM is able to trend CO over time, but it is not interchangeable with invasive or noninvasive measures. Use as a trending tool of CO in neonates likely requires initial and periodic calibration with echocardiography. Additional studies demonstrating adequate reliability are needed prior to independent use.
tracers. ASL-pMRI-estimated cerebral blood flow measurements in neonates undergoing hypothermia for perinatal hypoxic-ischemic encephalopathy correlate strongly with NIRS (r = 0.88) (29). ASLpMRI may be used to identify and quantify the severity of cerebral hyperperfusion after global cerebral anoxic brain injury, though at this stage, the technique is not useful as a bedside clinical tool to guide decision making.
when pulmonary artery pressure is suprasystemic and bidirectional (right to left in systole, left to right in diastole) when pulmonary artery pressure is approximately systemic. While a bidirectional ductal shunt is common in preterm infants on the first day of life, a persistent bidirectional ductal shunt has been associated with increased mortality, likely a surrogate marker of persistent PHT due to severe pulmonary disease. The restricted or “closing” pattern of a PDA depicts a veryhigh-velocity ductal shunt (peak systolic velocity >2.0 m/s) and high peak systolic-to-minimum diastolic velocity ratio (<2.0) (Fig. 29.2). A hemodynamically significant PDA is characterized by an unrestrictive or “pulsatile” left-to-right flow pattern, with the highest peak velocity at the end of systole and very low diastolic velocity. While peak systolic velocity less than 1.5 m/s has been traditionally described as “unrestrictive,” higher peak systolic velocities may be seen in infants with large unrestrictive ductal shunts, owing to very large shunt volumes or in the situation of a chronic funnel-shaped PDA, posttreatment, where there may be partial restriction at the pulmonary end.
signifying a predominance of early LV diastolic filling. The myocardium in preterm infants is less compliant resulting in impaired passive diastolic filling, reliance on atrial contraction for ventricular filling, and a resultant mitral valve E:A ratio less than 1. In the presence of a large ductal shunt, increased end-systolic left atrial pressure results in earlier mitral valve opening and a shortened IVRT (often <45 milliseconds) (33) and increased early passive filling velocity and flow, with E:A greater than 1, termed “pseudonormalization” (Fig. 29.2).
TABLE 29.2 Echocardiography Parameters of Ductal Hemodynamic Significance | ||||||||||||||||||||||||||||||||||||||||||||||||||||||||||||||||||||||||||||||||||||||||||||||||||||
---|---|---|---|---|---|---|---|---|---|---|---|---|---|---|---|---|---|---|---|---|---|---|---|---|---|---|---|---|---|---|---|---|---|---|---|---|---|---|---|---|---|---|---|---|---|---|---|---|---|---|---|---|---|---|---|---|---|---|---|---|---|---|---|---|---|---|---|---|---|---|---|---|---|---|---|---|---|---|---|---|---|---|---|---|---|---|---|---|---|---|---|---|---|---|---|---|---|---|---|---|
|
TABLE 29.3 Echocardiographic PDA Score at 48 Hours of Life in Preterm Infants Less Than 32 Weeks of GA and the Outcome of Death or Neurodevelopmental Impairment at 2 Years | |||||||||||||||||||||||||||
---|---|---|---|---|---|---|---|---|---|---|---|---|---|---|---|---|---|---|---|---|---|---|---|---|---|---|---|
|
the evaluation of many different testing kits, each with its own reference value range, rendering the interpretation of results more challenging. The early identification of infants at high risk for developing a symptomatic PDA may be clinically useful in centers seeking to administer targeted treatment aimed at early ductal closure. In very preterm infants, elevations in plasma BNP and NTpBNP concentrations at birth and on the first day of life correlate with lower gestational and birth weight, but not the development of PDA. After the 2nd day of life, elevated concentrations predict hemodynamically significant PDA (Table 29.4), though the interpretation of widely varying cutoff values of BNP and NTpBNP is hampered by the use of different testing kits and diagnostic criteria for symptomatic PDA. Martinovici et al. (43) found that plasma NTpBNP level less than 10,000 pg/mL measured on the 2nd day of life had 89% sensitivity and 100% specificity for spontaneous ductal closure.
TABLE 29.4 Relationship Between Plasma BNP/NTpBNP Concentration in the First 3 Days of Life and a Diagnosis of hsPDA in Preterm Infants | ||||||||||||||||||||||||||||||||||||||||||||||||||||||||||||||||||||||||||||||||||||||||||||||||||||||||||||||||||||||||||||||||||||||||||||||||||
---|---|---|---|---|---|---|---|---|---|---|---|---|---|---|---|---|---|---|---|---|---|---|---|---|---|---|---|---|---|---|---|---|---|---|---|---|---|---|---|---|---|---|---|---|---|---|---|---|---|---|---|---|---|---|---|---|---|---|---|---|---|---|---|---|---|---|---|---|---|---|---|---|---|---|---|---|---|---|---|---|---|---|---|---|---|---|---|---|---|---|---|---|---|---|---|---|---|---|---|---|---|---|---|---|---|---|---|---|---|---|---|---|---|---|---|---|---|---|---|---|---|---|---|---|---|---|---|---|---|---|---|---|---|---|---|---|---|---|---|---|---|---|---|---|---|---|
|
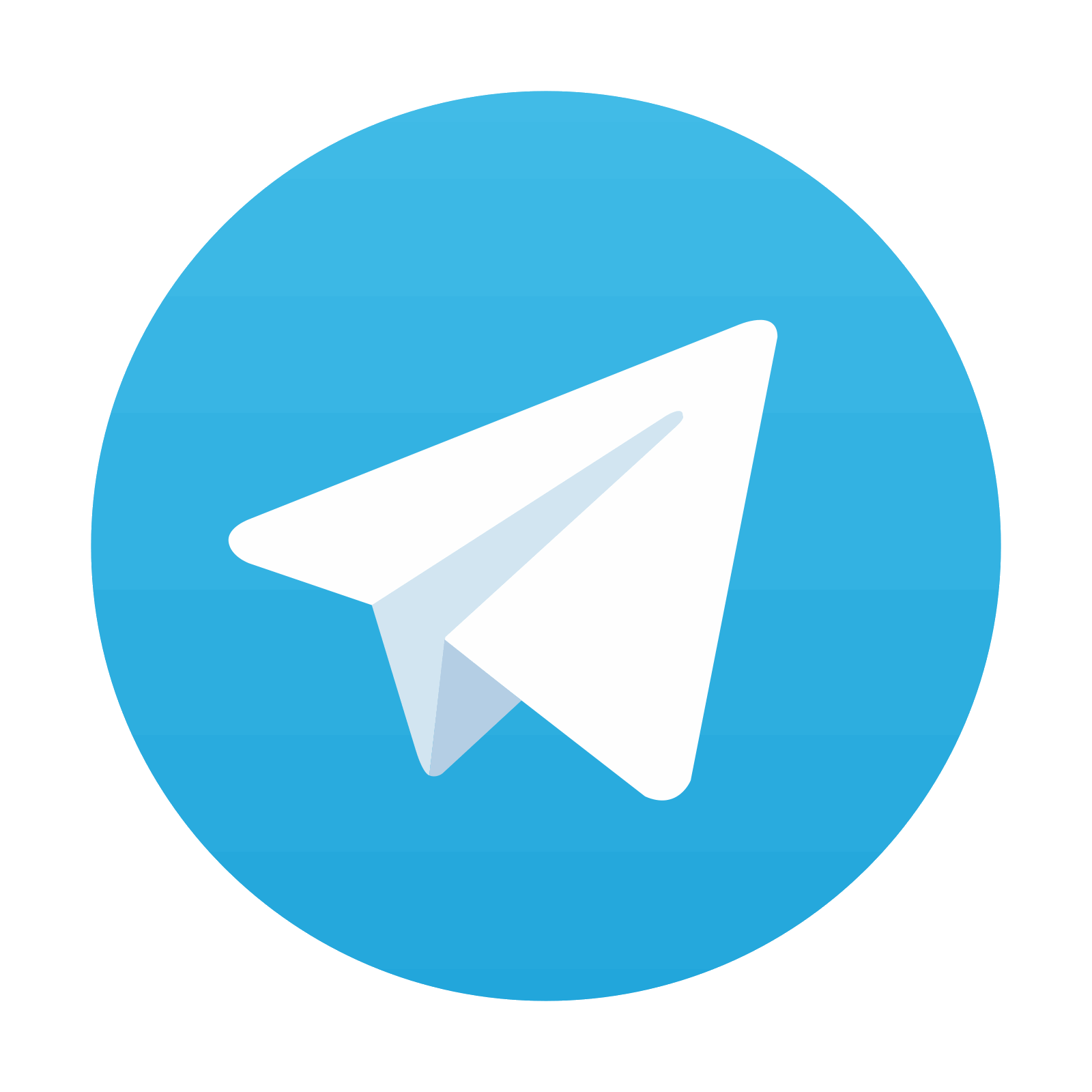
Stay updated, free articles. Join our Telegram channel
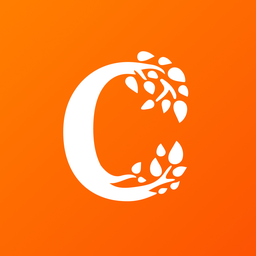
Full access? Get Clinical Tree
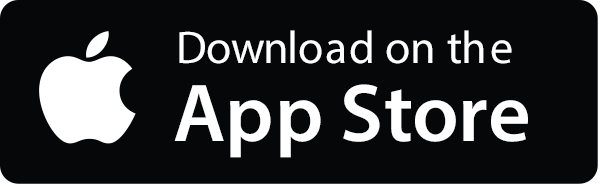
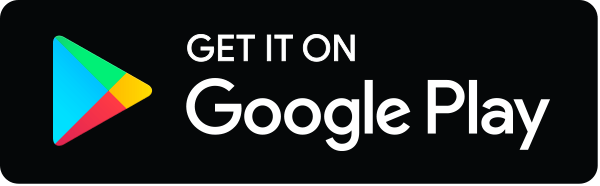
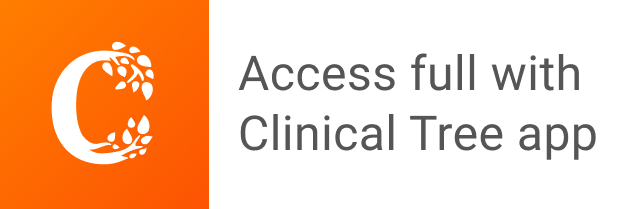