Functional Neuroanatomy of the Cerebrospinal and Autonomic Nervous Systems
Steven T. Tanaka
Charles J. Martin
FUNCTIONAL ANATOMY OF THE CEREBROSPINAL NERVOUS SYSTEM
From the neonate to the geriatric patient, a working knowledge of neuroanatomy and neurophysiology is helpful when examining the integrity of a patient’s nervous system. A neurologic evaluation then can be custom designed for each patient based on the presenting history. This is particularly valuable in wellness care because it helps the doctor monitor the integrity of the patient’s neuromusculoskeletal system throughout the course of his or her life. If abnormalities such as subtle neurological changes are found, then the structures that may be affected may be narrowed down in a systematic manner.
The “safety pin cycle” was a simple model used by early chiropractors to explain the physiologic effect of the chiropractic adjustment and subluxation on the health of the organism. Ongoing developments in modern neurosciences have shown that this model is overly simplistic, but it does not deny its basic concept of a feedback loop. It is a more accurate representation of physiologic processes in contrast with the orthodox, linear, cause-effect model that is still being used in mainstream health care. There are numerous additions that must be added to the cycle, such as neurotransmitters, multilevel synaptic connections, receptors, and other chemicals and structures. An interference in this cycle, such as that caused by a subluxation, may cause a disturbance in this system. It is important to consider the effects of an interruption in the normal flow of information among the growing cells and systems in a child, particularly noxious signals to the nervous system, and the ramifications that those noxious signals may have on the future development and health of the individual in light of the concepts of cellular memory, neural plasticity, and homeostasis/allostasis.
This is an overview of functional neuroanatomy to provide the doctor with a foothold to explore pediatric neurology. The second part of this chapter provides up-to-date information on the ever-complex autonomic nervous system (ANS). Chiropractors talk about the primacy of the nervous system in the health of an organism. Therefore, it is important that the doctor has some knowledge of the anatomy and physiology of the nervous system. The references used in this chapter will help the doctor expand his or her knowledge of the nervous system.
Postnatal Development of the Nervous System
Postnatal growth of the nervous system is greatest during the infant’s first 2 years. Most prominent is the growth of the brain. The brain grows two and one-half to three times its size at birth during the first year of life—it weighs about 350 grams at birth and grows to about 1,000 g by the child’s first birthday. There is marked proliferation of supportive structures, such as glial cells. Growth slows after this time. At puberty, the brain weighs about 1,250 g in girls and 1,375 g in boys (1). At birth, the brain exceeds 10% of the entire body weight compared with about 2% in the adult (1), and water constitutes about 85% of the brain’s weight postpartum, but by the age of 4, this figure drops to about 75% (2).
An active area of neurological research is how the growing nerves connect specific end organs in the periphery to the central nervous system (CNS). Current speculation on developing neuronal pathways centers on chemicals that repel or attract the growing neurons. As the neuron grows, it may be repelled by the surrounding tissues and alter its course until it finds the tissues with the attracting chemicals. This helps explain the individual variations in the course and area of innervation of the various nerves. There is a growth cone on the end of the immature peripheral motor nerve’s axon that leads it to its target. The process by which specific axons are able to branch off from the nerve bundle to go to their target muscle is unknown. The axons have been found to continue with the nerve bundle past their target muscle when a couple of recently identified proteins have been removed. A protein tells the nerve to stop at the target. Other proteins set up the neuromuscular connection (3). It is a marvel to consider how specific nerves connect end organs to the correct part of the brain and do this millions of times.
Myelination begins during the last trimester of pregnancy and continues after birth. Myelination of the spine begins in the cervical spine and progresses caudally (2). It also ascends to the brainstem and the brain prenatally and for a few months postnatally (4). Myelination of the ventral root occurs before that of the dorsal root (2). Myelination of most of the axons of the major spinal tracts and the cerebrum are largely completed by the end of the second year of postnatal life (5). The cerebrum completes its myelination from the anterior to the posterior (5). Full myelination is completed after the 10th year of life (5). An illustration of the myelination developmental process is that gray matter represents 35% of the matter in the cervical spinal cord of a newborn; in the adult, this is reduced to 19% when full myelination is completed (6).
At birth, the cerebellum continues to grow, with further cellular differentiation and migration and continued myelination. By adulthood, the surface area of the cerebellum has grown four-fold (6). Structures such as the reticular formation, commissural neurons, and intercortical association areas mature after the 10th year of life (5).
The dendrites of the cortical neurons are quite rudimentary at birth. During the first year of life, each cortical neuron develops 1,000 to 100,000 connections with other neurons (2).
At birth, the caudal end of the spinal cord is at the L3 vertebral level (2,6,7)—the cord is about 15 to 17 cm in length (2). By adulthood, the growth of the spinal column has moved the conus medullaris to the level of the inferior margin of L1, and the filum terminale has its cephalic end at the L2 level (6,7). This changes the angulation of the nerve roots exiting the intervertebral foramina.
In the neonate, the nervous system has yet to fully develop and integrate. This is apparently because of the presence of primitive reflexes. These signs may show up in later life when there is a high-level lesion or disorganization or degenerative changes. As each sign disappears in the infant, and as the infant is able to perform more tasks and control more of its physical and mental functions, the evaluator knows that there is increasing organization of the nervous system—the “wiring” is maturing. The integration usually occurs at a similar time in most infants, so charts have been developed that have chronologically recorded these “milestones.”
OVERVIEW OF THE PRIMARY STRUCTURES OF THE NERVOUS SYSTEM
The following is a brief review of the functional neuroanatomy of the central and peripheral somatic nervous system. When a lesion is suspected in the nervous system, the first step is to pinpoint the location(s) and/or structure(s) that is/are damaged or dysfunctional. This section focuses on the “somatic” portion of the nervous system. Because most readers will be fairly well acquainted with this portion of the nervous system, the description will be brief. After that will be a more in-depth description of the autonomic or visceral portion.
Cerebrum
The cerebrum includes the cerebral cortex, subcortical white matter, corpus callosum, basal ganglia, internal capsule, hippocampal formation, olfactory tracts and bulbs, and the diencephalon, that is, the thalamus, epithalamus, hypothalamus, and metathalamus. It forms most of the structures that we call the brain (Fig. 9-1). Also in the cerebral hemispheres are the cerebrospinal fluid (CSF)-filled lateral ventricles.
Cerebral Cortex
The cerebral cortex is characterized by its convoluted surface, which greatly expands the surface area in a container of limited size: the skull. An estimated 14 to 16 billion neurons reside in the gray matter of the adult cerebral cortex (8). Combining the number of neurons with the seemingly infinite number of potential and actual interconnections between dendrites and axons, the ability of the brain is difficult to comprehend.
The infantile reflexes and reactions (e.g., milestones) come and go as the cerebral cortex develops. A few examples include the optical righting reflex, grasp reflex, sucking and rooting reflex, plantar grasp reflex, and many others that are described in Chapter 11.
The neocortex, which averages 2.5-mm thickness, has six layers, or laminae, in its gray matter. Older cortical areas, such as the olfactory cortex, hippocampus, and dentate gyrus, have only three layers. The layers in the primary sensory cortex and motor cortex have poor differentiation. The motor cortex has large pyramidal cells in layers III and IV and seems diffuse compared to the sensory cortices, which have smaller, mainly granular-type cells that seem to be compacted (9).
The outermost cortical lamina (layer I) is the molecular, or plexiform, layer. It is composed primarily of dendrites. Many of the fibers come from the thalamus. The next layer (layer II) is the external granular layer. The granule and pyramidal neurons that are densely packed in this layer terminate in the molecular layer, whereas the axons pass into deeper cortical layers and the cerebral white matter. This layer is largely afferent. The third layer, the external pyramidal layer, is formed by pyramidal neurons and is mainly efferent. The dendrites are mainly in the molecular layer and the axons primarily pass through the cortical white matter as association or commissural fibers. The internal granular layer (layer IV) is largely composed of stellate and granule neurons. Most of the axons remain in this layer and many thalamic fibers terminate in this layer. The pyramidal layer (layer V) has pyramidal neurons and some granule neurons. The pyramidal neurons ascend and terminate in the molecular and internal granular layers. The pyramidal neuronal axons travel through the white matter as association or projection fibers to the basal ganglia, thalamus, other subcortical nuclei, limbic area, cerebellum, and spinal cord. The innermost layer, the multiform or fusiform layer, is filled with spindle-shaped, stellate, and granule neurons. The dendrites of these neurons ascend to the molecular layer, although most terminate in the internal granular layer (layer IV) or remain within the multiform layer. The fibers that ascend to layer IV communicate with the thalamic fibers that terminate in that layer. The axons of the spindle-shaped neurons are usually projection or association fibers. The stellate neurons are thought to be association fibers that connect gyri (9).
In addition to the horizontal passages, the cortex has vertical columns. Both the width and distance between minicolumns are greater on the left than the right hemisphere. There are more large pyramidal cells in the minicolumns in the left hemisphere than in the right. Macrocolumns appear to be the same size left and right (10). The columns contain thalamocortical neurons that terminate in the fourth layer, or lamina, onto the stellate cells. These then stimulate the pyramidal cells in the column in layer III, which then stimulate surrounding pyramidal cells. These in turn stimulate more neurons in adjacent columns and pyramidal cells in layer V. Other neurons in the surrounding area have inhibitory effects in a “surround inhibition” (11). Corticocortical from pyramidal cells in collateral areas terminate in layers II and IV. Layer II and III association fibers terminate in the ipsilateral hemisphere in layer II (11).
The cortex has gyri with the infolding sulci. Current thoughts on what causes the gyri and sulci and fissures are the number of and forces exerted by the developing
neurons. The dendrites anchor the neuron for the cell body and axon. When there are a significant number of neurons that exert a sufficient amount of force, in particular when there is a high concentration of neurons, a gyrus is forced. When there are fewer neurons and less force, the sulci and fissures are formed. In dense, inter and intraconnected regions such as the motor and sensory cortices, these areas are prominent. In addition, the amount and type of pressure on the neurons affects their shape. Ones that have lateral pressure, such as those in gyri, are elongated. Others that are in sulci may be stretched and appear flattened (12). Some current researchers are using functional magnetic resonance imaging to look for differences in the brain structure between “normal” brains and those of people with autism, schizophrenia, dyslexia, and other disorders associated with the brain. Preliminary studies have found some structural differences, particularly with the location and size of the gyri and sulci. Another area of research is to see if there are cortical differences among those with certain talents, such as a musician versus a non-musician or between those who play different instruments (12).
neurons. The dendrites anchor the neuron for the cell body and axon. When there are a significant number of neurons that exert a sufficient amount of force, in particular when there is a high concentration of neurons, a gyrus is forced. When there are fewer neurons and less force, the sulci and fissures are formed. In dense, inter and intraconnected regions such as the motor and sensory cortices, these areas are prominent. In addition, the amount and type of pressure on the neurons affects their shape. Ones that have lateral pressure, such as those in gyri, are elongated. Others that are in sulci may be stretched and appear flattened (12). Some current researchers are using functional magnetic resonance imaging to look for differences in the brain structure between “normal” brains and those of people with autism, schizophrenia, dyslexia, and other disorders associated with the brain. Preliminary studies have found some structural differences, particularly with the location and size of the gyri and sulci. Another area of research is to see if there are cortical differences among those with certain talents, such as a musician versus a non-musician or between those who play different instruments (12).
The major division of the cortex is the lobes. They are named after the osseous structures that overlie them. The anterior portion is the frontal lobe, which is separated from the middle region, the parietal lobe, by the fissure of Rolando. The temporal lobe is separated superiorly by the fissure of Sylvius and by an artificial posterior line from the occipital lobe. An artificial line separates the parietal lobe from the occipital lobe.
![]() FIGURE 9-2 A: Motor homunculus. B: Sensory homunculus. Reprinted from Nolte J. The Human Brain (3rd ed). St. Louis, MO: Mosby Year Book, 1993, with permission. |
Knowledge of the particular function of each cortical area is invaluable when localizing suspected cortical lesions. The precentral gyrus deals with motor control. Posterior to that is the postcentral gyrus, or somatosensory cortex. Together, they form the primary sensorimotor cortex. The sensorimotor cortex is somatotopically organized, that is, the medial aspect of these regions, which is located cephalically and within the central fissure, controls the lower extremities and the lateral aspect and is associated with progressively higher regions of the body—this is the well-known “homunculus.” Although the “homunculus” is a valuable aid during localization, modern neurobiologists have found that the areas representing each region of the body in the motor cortex are not distinct as is classically illustrated. Each neuron has an influence over various muscles because movements usually involve multiple muscles and joints rather than a one-to-one relationship between neurons and muscles (Fig. 9-2). The homunculus is, in a word, “fuzzy.” The posterior aspect, the occipital lobe, is associated with vision. There are also association areas that integrate sensory inputs and motor responses. The temporal lobe is located in the inferolateral region of the cerebral cortex and is separated from the upper regions by the lateral cerebral fissure, or fissure of Sylvius, and functionally from the posterior regions. The hippocampal and parahippocampal regions are prominent parts.
The insula is located deep within the fissure of Sylvius. The major activities of the temporal lobe are speech, auditory association, and memory.
The insula is located deep within the fissure of Sylvius. The major activities of the temporal lobe are speech, auditory association, and memory.
Cortical asymmetry is found in many animals, but it is thought that the particular asymmetries and their functions in humans may be unrelated to the hemispheric differences in other creatures. Although hemispheric specialization probably existed before man and other animals have hemispheric specialization, human hemispheric specialization followed a different path by replacing function of a section of brain with different tasks that better serves human beings. It is thought that each hemisphere took control of certain situations. The right might have developed to control potentially dangerous situations that required quick reactions, that is, more “below-up” or externally motivated behavior. The left hemisphere seems to be involved in “above-down” self-motivated behavior (13).
An example of asymmetrical cortical control for functions is speech. Knowing where this area is located aids in localizing some cortical lesions. An example is Broca’s, or expressive, aphasia, which is associated with a lesion to Brodmann’s area (BA) #44, or the motor speech area in the left inferior frontal lobe.
Right-handedness is more common than lefthandedness. Nature abounds with right side-left hemisphere dominance. Many animals, such as reptiles and birds, tend to strike prey with the right side after observing their target with the right eye (13).
The association and commissural fibers connect different areas of the cortex. They are the primary components of the white matter of the cerebral hemispheres (14). The afferent and efferent connecting fibers between the cortex and other central structures—that is, brain stem and spinal cord—form the corona radiata. These fibers converge to form the internal capsule as they pass between the thalamus and caudate nucleus and the lentiform nuclei (8).
Other components of the cerebrum include the commissures, principally the corpus callosum, anterior commissure, and hippocampal commissure, which interconnect the cerebrum’s different parts, the ventricles, and the internal capsule.
Major Regions of the Cerebral Cortex Brodmann came up with a map of the brain with 47 areas based upon the cytoarchitecture of each part. Further studies of the brain and modern technological tools that observe the functioning brain, such as positron emission tomography and functional magnetic resonance imaging, have found that the areas are not as distinct in function, with some crossover regions in the margins and between areas. The following are primary and secondary selected Brodmann’s areas of the brain.
Frontal Lobe This is the forwardmost aspect of the cerebral cortex and forms one-third of the lateral surface of the brain. It has distinct boundaries. The posterior border is the central sulcus, or fissure of Rolando, separating it from the parietal lobe and inferiorly by the lateral cerebral fissure, or fissure of Sylvius, which separates it from the temporal lobe. On the medial surface of the hemispheres its border is the superior longitudinal fissure.
The frontal lobe provides cognition of motor intentions, and the parietal lobe provides tactile and visuospatial. It is often active bilaterally. It is active when one sees, feels, or otherwise senses an object and then reaches for it or, if it is not in sight, identifies the object one manipulates. It projects to the brainstem nuclei associated with the reticulospinal tract (15). The prefrontal cortex is associated with executive functions such as reasoning and judgment. The posterior aspect in the precentral gyrus laterally and part of the paracentral lobule medially is the motor cortex.
Parietal Lobe Anterior, on the lateral side of the hemispheres, the parietal lobe begins at the central sulcus and extends posteriorly and posteroinferiorly to an indistinct border with the occipital and temporal lobes at the level of the lateral cerebral fissure. On the medial side of the cerebral cortex, the parietal lobe extends posteriorly to the parieto-occipital fissure and, like the frontal lobes, its inferior border is the superior longitudinal fissure. On the anterolateral aspect are BAs #3, #1, and #2 (primary somatosensory cortex). Behind these areas are BAs #5 and #7 in the superior aspect of the parietal lobe and BAs #39 and #40 in the inferior. In the inferior region are the supramarginal (BA #40) and angular gyri (BA #39) (these two gyri will be discussed later).
Occipital Lobe It is posterior to the parieto-occipital fissure and sits atop the tentorium cerebelli. It is subdivided into the superior and inferior gyri by the transversely running lateral occipital sulcus on the lateral surface and into the cuneus (BA #17) and lingula gyrus on the inferior surface. It is the visual center of the brain. Visual signals from the retina pass through the optic tracts to the lateral geniculate bodies in the thalamus. They then pass to the visual cortex.
The primary visual or striate cortex (BA #17, or V1) is involved in conscious vision. The secondary visual cortex (BA #18), or peristriate area, communicates with the primary visual cortex and BA #18. It interprets, associates, and facilitates visual images from the primary visual cortex and has connections to the contralateral cerebral hemispheres, the prefrontal cortex, and the parietal lobe (16).
The cuneus receives visual input that views the inferior visual field via the contralateral superior
retina. The lingula receives visual impulses from the contralateral inferior retina, which covers the superior visual field.
retina. The lingula receives visual impulses from the contralateral inferior retina, which covers the superior visual field.
Temporal Lobe The temporal lobe is inferior to the lateral inferior cerebral fissure, or fissure of Sylvius, and extends posteriorly toward the occipital and parietal lobes. Olfactory and auditory processing occur in the temporal lobe, as does memory processing. Wernicke’s area (BA #22) is located on the left temporal lobe. Heschl’s gyrus, or the primary auditory area (BA #41), is also located in the temporal lobe.
Insula, or Isle of Reil The insula is now known as the fifth lobe of the cerebral cortex. It is found by spreading apart the opposing surfaces, or operculum, of the lateral cerebral fissure. A central sulcus divides the insula into two gyri. There are gyri in front of and behind the central sulcus. It communicates with the frontal and parietal lobes. It also connects to the temporal lobe and has extensive connections within itself (17). It has visceral sensory and motor and somatic sensory and motor functions. Autonomically it is involved in gastrointestinal and cardiac regulation. It is also a motor association area (15,16). It aids memory related to language. It also serves as an olfactory gustatory correlation area (17).
Prefrontal Cortex (BA #9) It has bidirectional communications with the neocortex bilaterally but not with the primary motor and sensory areas. The human prefrontal cortex is large compared with the sizes of the prefrontal cortex in other creatures. One may say that the prefrontal cortex is what makes humans human because it deals with “executive functions.” These high functions of the brain include abstract thinking, decision making, and social behavior (18). It is important in conscious learning. It connects movement to behavior, as is described in Chapter 11. The prefrontal area is also engaged in short-term, “working” memory (19). The prefrontal cortex is well connected to the limbic system.
Hippocampal Formation This is formed by the parahippocampus, hippocampus or Ammon’s horn, and dentate gyrus and it is a part of the limbic system deep within the cerebrum. It is involved in memory and learning. Hypoglycemia, anoxia, and status epilepticus may cause severe hippocampal cell loss (20). The hippocampal commissure interconnects the hippocampal formation.
The hippocampus is bilateral and is located in the medial aspect of the temporal lobe. Afferent supply is primarily from the entorhinal cortex and is its major output. It has been associated with long-term memory and spatial navigation. It is an early region affected by Alzheimer’s disease. Lesions have also been associated with amnesia. Temporal lobe epilepsy often causes visible damage to the hippocampus. Long-term taxi drivers have been found to have larger posterior regions of the hippocampi. It is an area that, more than other areas of the brain, has a high rate of neurogenesis that continues into adulthood. Researchers find that continual learning, especially of complex tasks, throughout life stimulates this neoneurogenesis (21). Because it is in the older part of the brain, unlike the neocortex, the hippocampus has three layers: molecular, pyramidal, and polymorphic layers.
The hippocampus seems to be vulnerable to corticosteroid use. Studies have found hippocampal atrophy with the use of corticosteroids. Outward manifestations are memory impairment and depression (22).
Like the hippocampus, the dentate nucleus has three layers: molecular, granular, and polymorphic. Its neurons remain inside the hippocampal formation (18). The parahippocampal, or entorhinal, region (BA #28) forms most of the parahippocampal gyrus. It receives its afferent supply from association areas in the cerebral cortex and from the olfactory stria. It is the principle path between the cerebral cortex and the limbic part of the hippocampus (20).
Cingulate Cortex It is a part of the limbic system and surrounds the corpus callosum. It receives afferent information from itself, bilaterally, and from the anterior thalamic nuclei and temporal lobe. It projects to the corpus striatum and subcortical limbic nuclei. It is part of the circuit of Papez (see the section about the thalamus later in the chapter for more about the Papez circuit).
Sensory Areas Somatosensory or Somesthetic Cortex (SI; BAs #1,#2, and #3). It is located in the postcentral gyrus laterally and the posterior paracentral lobule medially. Pain and thermal sensations may be shared with the frontal lobes to compare the quality of the sensation and create relevant actions in which to engage. It also fields visceral pain, which is diffuse and difficult to localize after being processed by the thalamus (23). BA #3 is subdivided into separate areas: #3a, which is the terminus for muscle spindle impulses, and #3b, which is the cutaneous receptor area and the “true” somatosensory cortex. Like the motor cortices, there is a sensory version of the inverted homunculus. The somatosensory cortex receives afferents from the opposite somatosensory cortex via the corpus callosum and association neurons from the primary motor cortex, many of which are from the corticospinal tracts. Most of the afferent supply that enters the efferent information in association fibers travel to BA #5, the somatic sensory association area, as well as BA #40, the somatosensory association area, which is in the inferior parietal cortex. Commissural neurons travel to the opposite
somesthetic cortex, and projection neurons pass through the pyramidal tract to the ipsilateral ventral posterior nucleus of the thalamus and to the spinal cord in the contralateral posterior column and spinal dorsal gray horn (24).
somesthetic cortex, and projection neurons pass through the pyramidal tract to the ipsilateral ventral posterior nucleus of the thalamus and to the spinal cord in the contralateral posterior column and spinal dorsal gray horn (24).
In addition to the afferent supply from the various aforementioned sources, the connectors in the various cortical layers intercommunicate extensively. The interconnections allow for the sharing of receptive fields as well as to the specific task of each area (23).
Visual, or Striate, Area (BA #17) It is located in the calcarine sulcus on the margins of both the cuneus and lingual gyri of the occipital lobe.
Auditory Area (BAs #41 and #42) It is in Heschl’s gyrus of the temporal operculum of the superior temporal gyrus.
Gustatory Area (BA #43) It is located on the ventral portion or opuculum of the postcentral gyrus.
Primary Olfactory Area It is in the allocortex of the pyriform and periamygdaloid regions. Unlike many of the other sensory terminations, the impulses do not pass through the thalamus but go directly to this area.
Motor Areas
Primary Motor Cortex (BA #4) It is located on the anterior wall of the central sulcus and adjoining portions of the precentral gyrus of the frontal lobe on the lateral surface and the anterior portion of the paracentral lobule. It constitutes 60 to 80% of the pyramidal tracts and is the primary origin of the decussating corticospinal tracts (18). There is somatotopic representation (inverted “homunculus”) of largely the opposite side of the body. Its afferent supply comes via the corpus callosum from the opposite motor cortex, the somatosensory cortex (BAs #3, #1, and #2), the contralateral dentate nucleus of the cerebellum, and the supplementary motor area (18). The majority of its afferent nerve supply is from the ventral lateral thalamic nucleus.
About one-third of the nearly one million neurons in the pyramidal tract arise from the primary motor area; almost another one-third comes from the premotor cortex, and about 40% come from the parietal lobe: BAs #3, #1, #2, #5, and #7 (25).
Premotor Cortex (BA #6) It is located on the lateral and medial surfaces of the cerebral hemispheres, posterior to the primary motor cortex in the most posterior section of the frontal lobe. It is six times larger than the primary motor cortex. Input comes from BA #3 and #7 (somatosensory areas). It is an extrapyramidal motor area (26). Fibers from sources such as the somatosensory auditory and visual association cortices, the primary somatosensory cortex, and the ventral lateral nucleus of the thalamus send out projections to the motor cortex, basal ganglia, and spinal cord (25). Among the functions of the premotor cortex are cortical automatic associated movements or movement patterns that accompany fine, skilled, voluntary movements. An example is the strong grasp reflex seen for a time among infants (26). Part of the premotor cortex, primarily on the medial hemisphere, is the supplementary motor area. Once a sequence of motor activities is learned, the automated sequence can be done with activation of the supplementary motor area. As you are learning a task, such as manual spinal adjustments, a more lateral area of the premotor cortex is engaged. Once a manual skill is mastered and familiarized, the supplementary motor area is activated when the task is being done. For example, once one can do a particular manual adjustment, the set-up can pretty much be done without tremendous effort. On the medial side of the cerebral hemispheres, in BA #6, there is the supplementary motor area. Using internal cues, it is associated with motor planning and intent to move. It seems to preprogram sequences of movements that are in motor memory (18). This is an important area to develop as a child and to engage during any conscious task, and it is obviously important to the chiropractor when learning to perform adjustments.
Association Areas
Somatosensory Association Cortex (BA #5) It is involved in hand-eye coordination, such as visually observing yourself reaching for something with your (contralateral) arm/hand (18).
Inferior Parietal Cortex and Supramarginal Gyrus (BA #40) This is an important area for chiropractors. It integrates tactile, muscular, articular, and kinesthetic afferents in BAs #3, #1, and #2, as well as from BAs #7 (visual input), #5 (tactile input), and #23 (posterior cingulated cortex for limbic input). It provides body awareness. A major function is stereognosis, the ability that allows one to identify objects manually, such as during manual digital palpation. BA #40 on the right side helps with physical identification of unseen objects, whereas the left hemispheric BA #40 aids in functional identification (18). In the infant and child this area requires constant stimulation. An environment rich in objects to handle and manipulate obviously helps this area develop. All parents identify the need for infants and children to reach for any and all objects, often to their consternation, which causes them to hide dangerous objects—the fuzzy toy is okay; that knife is not okay and must be taken away and hidden.
Secondary Somatosensory Cortex (SII or BA #43) This region on the medial surface of the parietal operculum of the insula receives nociceptive input from the thalamus. The output goes to the primary and premotor cortices (27). SI and SII together may be associated with tactile discrimination (18).
Asymmetrical Areas There is asymmetry in the cerebral hemispheres. Left brain-right brain differences are common knowledge. The left brain is considered to be more analytical, whereas the right brain is the “artistic” side that processes shapes and spatial orientation. With respect to the auditory association areas, the right side “hears” music while the left side “hears” words.
Broca’s Area (BAs #44 and #45) is a motor speech area in the left inferior frontal gyrus. It is associated with speech but does not cause movement of the vocal elements. It coordinates or programs the movements needed to speak. Lesions cause apraxia or Broca’s aphasia (28). Wernicke’s Area (BAs #22 and #42, posterior region) in the left superior temporal gyrus is the region for understanding the spoken word. Its superior aspect is called the temporal plane. This area of the left temporal lobe is larger than its counterpart on the right side. The arcuate fasciculus ties together Broca’s area, Wernicke’s area, and the angular gyrus (28).
The angular gyrus (BA #39) of the inferior parietal lobe is often considered to be a part of Wernicke’s area and receives input from the lingual gyrus (BA #19) and then passes impulses on to the temporal plane. It is an area that stores words, syllables, and numbers or other symbols that are retrieved by visual cues—language symbolism. It is important in reading (converting the written syllables to sounds) and when listening to spoken word (18). Lesions may cause an inability to read and write without disturbing speech or the ability to understand speech, and they may cause an inability to name an object that one sees (27). The angular gyrus is considered by many to be the most important cortical area for speech and language (28). It is an area that develops quickly in the young child.
Basal Ganglia
The basal ganglia are composed of five subcortical gray matter nuclei: the caudate nucleus, putamen, globus pallidus or paleostriatum, claustrum, and the amygdala (29). Some authors include the subthalamic nucleus and substantia nigra (30). They are functionally related structures. The caudate nucleus, putamen, and globus pallidus form the corpus striatum. The caudate nucleus and putamen are called the striatum or neostriatum (31). The lentiform nucleus is composed of the putamen and globus pallidus.
The caudate nucleus is one of the C-shaped structures in the brain and borders the lateral ventricles. It curves along the ventricle until it joins the amygdaloid body posteriorly (30). The putamen is continuous with the ventral head of the caudate nucleus and also is joined to the internal capsule (30).
The globus pallidus has myelinated fibers, unlike the caudate and putamen. A lamina separates it into a lateral, or external, section, and a medial, or internal, segment. Laterally, it is separated from the putamen by the external medullary lamina. Together, the globus pallidus and putamen appear lens-shaped and hence its name: lentiform nucleus (30).
The claustrum is a thin band of fibers that is lateral to the putamen, from which it is separated by the external capsule of the putamen. The external capsule also separates it from the insular cortex. Fibers from it join the temporal lobe (30).
The substantia nigra is a dark brown color because of neuromelanin, a waste product of catecholamine metabolism. Of the three catecholamines within it, the best known is dopamine (30).
The subthalamic nucleus is a biconcave, lens-shaped structure that borders the internal capsule, hypothalamic sulcus, hypothalamus, and third ventricle. Functionally it is part of the basal ganglia, but structurally it, along with the zona incerta and nuclei of perizonal fields of Forel, is part of the subthalamus (30).
The basal ganglia communicate with the cerebral cortices, thalamus, subthalamus, ventral tegmental area, and substantia nigra (31). The ventral tegumental area and substantia nigra secrete the neurotransmitter dopamine. Malsecretion is often associated with basal ganglia dysfunction (31).
The basal ganglia form what is known as the extrapyramidal motor system. They are part of the feedback loops between the cerebral cortex and thalamus (31). They smooth movements and help transition from one type of movement to another. The classic dysfunction of the basal ganglia in these loops is Parkinson’s disease, which is caused by degeneration in the substantia nigra and depletion of dopamine and has a significant effect on the striatum (31). Huntington’s disease is associated with the efferent projections from the striatum to the globus pallidus and substantia nigra via the striatopallidal and striatonigral pathways that use γ-aminobutyric acid for transmission (31).
The basal ganglia integrate complex motor activities into previously learned behavior performance (32). In children, a lesion of the basal ganglia may cause Sydenham’s chorea, a disorder often associated with
rheumatic fever (33). Children with a familial history of Huntington’s chorea may become victims of this disease in their 30s or 40s, which affects the cerebral cortex and basal ganglia (33).
rheumatic fever (33). Children with a familial history of Huntington’s chorea may become victims of this disease in their 30s or 40s, which affects the cerebral cortex and basal ganglia (33).
Various other movement disorders are associated with the basal ganglia. Athetosis presents as slow, irregular, writhing movements that usually affect the distal upper extremities. When facial muscles are affected, there is grimacing and abnormal tongue movements. These are found in cerebral palsy, when the basal ganglia—in particular, the striatum—are involved. Ballismus appears as a sudden throwing motion of an extremity and is associated with the subthalamus. The best known dysfunction of the basal ganglia is Parkinson’s disease, which is caused by degeneration of the pars compacta of the substantia nigra. There it causes depletion of the neurotransmitter dopamine, which influences the striatum and the striatothalamic and thalamocortical pathways (31).
The basal ganglia have been found to have nonmotor functions. They have connections with the prefrontal and limbic regions of the cerebral cortex. They seem to influence cognition, language-specific functions, and emotions. They play a major role in developing a sequence of behavior into useful activities and have been called “cognitive pattern generators.” In other words, it turns thoughts into integrated actions (34). Many psychiatric disorders are associated with pathways that travel in and out of the basal ganglia and others with the neurotransmitter γ-aminobutyric acid, serotonin, and dopamine (28).
Thalamic Structures
The thalamic structures are located in the diencephalon and contain many nuclei. Thalamic structures are involved in the integration of neural impulses and secretion of many of the neurohormones.
Thalamus The thalamus is a relay station that is composed of at least 30 nuclear groups that integrate the sensory impulses other than those of olfaction. Auditory, vestibular, visual, and somatosensory signals pass through it (35). It is also the major source of afferent impulses traveling to the cerebral cortex (36,37). Afferent projections to the thalamus come from a variety of other sources, which include peripheral input from the spinothalamic and trigeminothalamic tracts, brainstem, cerebellum, and other thalamic structures (37). Efferent impulses from the thalamus project to the cerebral cortex, cerebellum, striatum, and other thalamic structures (37). It processes sensory information, such as hearing, vision, proprioception, and exteroception (38). Emotions and pain perception are associated with the thalamus (36).
The anterior thalamic nuclei are elements in the “emotional circuit of Papez.” Impulses from the hippocampus pass through the fornix and the mammillary body to the thalamus before continuing on to the cingulate gyrus. Impulses continue on to the parahippocampal gyrus before returning in a feedback loop back to the hippocampus. This circuit seems to be involved in short-term memory (36). The circuit of Papez and the “limbic system” or “visceral brain” are controversial and researchers are still trying to understand them and the structures involved.
Subthalamus The subthalamus is a structure that lies anterior and caudal to the thalamus and is adjacent to the internal capsule and hypothalamus. It is composed of the subthalamic nuclei zona inerta and nuclei of the tegmental fields of Forel (8). Its function is vague, but it seems to have excitatory influence on its targets. It is part of the extrapyramidal system (8).
Epithalamus The epithalamus is a mysterious area. It is composed of the habenular nuclei, the pineal gland, and the habenular and posterior commissures (37). It borders the posterior aspect of the third ventricle.
The pineal gland, or epiphysis, is a tiny structure that secretes melatonin, among other hormones. The quantity of melatonin that is secreted has been associated with the amount of light that the organism receives and amount of stress that the organism perceives. Science is constantly speculating about or finding new effects of melatonin in the body (36,38). An example of a structure that stimulates the pineal gland is the superior cervical ganglion. Norepinephrine produced by the ganglion stimulates receptors in the pineal gland to produce melanin. The pineal gland is partially calcified in the adult brain, which makes it a landmark in plain skull radiography (35).
The habenula is associated with autonomic responses to olfaction. The habenula is attached by three connections: stria medullaris thalami, habenular commissure, and habenulointerpeduncular fasciculus (36,38).
Hypothalamus The hypothalamus is part of the diencephalon and surrounds the third ventricle. There are anterior, middle, and posterior regions. The subependymal gray connects the hypothalamus to the rest of the CNS (38). There are approximately 30 nuclei in the hypothalamus. It controls the ANS. The hypothalamus is associated with responses to emotions and the maintenance of homeostasis. Hunger, fullness, and thirst are expressions of the hypothalamus (36). Many of the neurohormones produced in the hypothalamus are produced in a portion called the hypophysis cerebri, or pituitary gland (36).
The hypothalamic-pituitary-adrenal (HPA) axis aids in the maintenance of homeostasis. Maintaining homeostasis involves coordination of, in particular, the nervous, immune, and endocrine systems. In response to stress, corticotrophin-releasing factor is released by the paraventricular nucleus (PVN) of the hypothalamus to the anterior pituitary gland. This causes adrenocorticotrophic hormone to be released into general circulation. When it reaches the adrenal gland, glucocorticoids are synthesized and secreted from the zona fasciculi. The glucocorticoids find intracellular receptors to effect the activities of the HPA axis and create a major inhibitory feedback loop to the HPA axis. This is a simplification of the HPA axis, but it is the primary circuit.
There is a hypothalamic feedback loop that is associated with the regulation of hunger when serum glucose and other needed nutrients are low. The limbic system is important to the stress response. The hippocampus has an inhibitory effect to reduce the HPA reactions to stress. The prefrontal cortex releases catecholamines during the stress response and inhibits the HPA axis. The amygdala seems to stimulate the HPA axis, with the medial nuclei responding to “emotional” stress and the central nuclei to physiological stress. Stress stimuli cause the locus coeruleus to release norepinephrine and trigger the release of numerous chemicals associated with stress. As a result, the locus coeruleus is thought to affect emotions, behaviors, memory, and other types of responses to stress. Altered response by the HPA axis can have negative health consequences (39).
Glucocorticoid treatment and Cushing’s syndrome have been associated with alterations of the HPA axis. This can manifest with depressive symptoms and mood changes (40). Psychological stressors and cortisol release also have been implicated in alteration of the HPA axis and depression among both adults and children (41).
Amygdala
The amygdala, or amygdaloid complex, is on the temporal lobe near the lateral ventricle. Classically, it is associated with fear, uncertainty, and the unfamiliar. The nuclei within the amygdala are highly interconnected. There is considerable efferent outflow to the midbrain and brainstem. This activates rapid responses to danger. There are projections from the amygdala to the cerebral cortex and subcortical regions. The cortical and subcortical regions reciprocate with considerable input back to the amygdala (42). There is olfactory input (8), which may cause what is known as the “smell of fear.”
The amygdala has been found to be associated with perception, particularly with an emotional filter. Enlargement of the amygdala has been found with unusual or unique perceptions related to psychotic disorders. If one frequently uses circuits associated with emotions, that is, “exercises them,” then one’s perceptions may be more easily influenced by emotions. Frequent use may enlarge the parts being used frequently, in this case, the amygdala. Among the normal population, amygdala volume has been associated with different personalities (43).
Cerebellum
The cerebellum, or “little brain,” resides in the posterior fossa (44). Although it forms only 10% of brain volume, more than half of the neurons in the brain are located within the cerebellum (45). To enable it to do its work, a massive amount of information enters the cerebellum. There are forty times more axons projecting into the cerebellum than exiting it (45).
To do its work, the cerebellum communicates with the rest of the CNS via three peduncles on its anterior surface that attach to the pons level of the brainstem: the inferior, or restiform, body; the middle, or brachium, pontis; and the superior, or brachium, conjunctivum. Most afferent signals enter the cerebellum through the inferior and middle cerebellar peduncles. All efferent output exits via the superior cerebellar peduncle (38,46).
The inferior cerebellar peduncle is the passage for proprioception and other somatosensory afferent signals. These include the dorsal (trunk and extremities) and anterior spinocerebellar (spinal arcs), cuneocerebellar, reticulocerebellar, and olivocerebellar tracts. All but the olivocerebellar tract from the premotor and motor cortices via the olivary nuclei are ipsilateral or uncrossed before entering the cerebellum. Efferents from the flocculonodular lobe and the fastigial nuclei exit the cerebellum through the inferior peduncle, and these neurons are associated with the vestibular and reticular systems (46,47).
Entering through the middle cerebellar peduncle, the largest of the peduncles, are the pontocerebellar tracts, which are the primary pathways for efferent messages from the cerebral cortex. These cortical fibers synapse and largely decussate or cross over in pontine nuclei before traveling to the cerebellum. An interesting note is that fibers from the prefrontal cortex travel to pontine nuclei and then continue on to the cerebellum. These are higher-order signals that may be associated with behavior and cognition (46).
The ventral spinocerebellar tract passes into the cerebellum through the superior cerebellar peduncle (46). Visual, auditory, and vestibular senses in the tectocerebellar nerves pass through the superior peduncle (47). As noted above, most of the efferent neurons of the cerebellum pass through the superior peduncle.
Anatomically there are three distinct functional regions: a cerebellar cortex of gray matter, an internal
region of white matter, and three pair of nuclei (fastigial; interposed, which are composed of the globose and emboliform; and dentate). Three pair of tracts connect the cerebellum to the brainstem: the inferior, middle, and superior cerebellar peduncles (45). Afferent impulses enter the cerebellum from the spinal cord through the middle and inferior cerebellar peduncles. Much of the input to the cerebellum comes from the pontine nuclei (38). Most of the efferent impulses leave through the superior cerebellar peduncle (38). The rest of the efferent impulses pass from the flocculonodular or vestibulocerebellar lobe to the lateral and medial vestibular nuclei in the brainstem and control balance and eye movements (45). The flocculonodular lobe is phylogenetically the oldest part of the cerebellum (46).
region of white matter, and three pair of nuclei (fastigial; interposed, which are composed of the globose and emboliform; and dentate). Three pair of tracts connect the cerebellum to the brainstem: the inferior, middle, and superior cerebellar peduncles (45). Afferent impulses enter the cerebellum from the spinal cord through the middle and inferior cerebellar peduncles. Much of the input to the cerebellum comes from the pontine nuclei (38). Most of the efferent impulses leave through the superior cerebellar peduncle (38). The rest of the efferent impulses pass from the flocculonodular or vestibulocerebellar lobe to the lateral and medial vestibular nuclei in the brainstem and control balance and eye movements (45). The flocculonodular lobe is phylogenetically the oldest part of the cerebellum (46).
The cerebellum is best known as the center for regulating fine movements, muscle tone, and posture (32). Dysfunction or lesion degrades movement (32). Ataxia and dysmetria (overshooting or undershooting movements); hypotonia (flaccidity of the muscle with diminished resistance to passive limb manipulation related to diminished gamma and alpha motor neuron activity); and equilibrium and gait disturbances are classic signs of cerebellar lesions (48). Lesions of the cerebellum are also associated with asthenia (mild weakness), atonia (diminished resistance to limb manipulation), and astasia (jerky movements) (49).
A commonly recognized effect of cerebellar lesions is ataxia. Alcoholic drunkenness can cause a form of cerebellar ataxia that affects the vestibulocerebellar pathway. When someone is apprehended for public drunkenness, particularly while driving a motor vehicle, the tests done evaluate cerebellar function. These include tandem walk (walking a straight line by putting one foot in front of the other) and the dysmetria test of having the arms outstretched and attempting to touch the fingertips to the nose but over or undershooting. Sometimes during this procedure, there may be intentional tremors when the sign is due to cerebellar lesion.
Nystagmus may be caused by cerebellar lesions as well. There may also be gaze instability that causes diplopia. Cerebellar lesions may cause dysarthria. Speech is often halting, uneven, irregular, poorly modulated, explosive, or scanning (46).
There is a growing body of research stating that the cerebellum has functions beyond the management or “fine-tuning” of movement, that is, beyond motor-only functions. Recently, researchers have shown that the cerebellum influences cognition and behavior. Lower intellect and neuropsychiatric or behavioral disorders have been found in those with cerebellar dysgenesis or dysfunction, often while the rest of the brain seems to be normal (50). Researchers have consistently found abnormalities in the cerebellum of those with early infantile autism. Because there are often abnormalities found in other parts of the nervous system, in particular the limbic system, it is difficult the ascertain the influence of cerebellar abnormalities on autism (50). Research has found that the cerebellum is part of the circuitry for learned conditioned responses and high-level skills (50). Sudden severe damage to the cerebellum, usually caused by trauma or thrombosis, with resultant cerebral functional deficits cause cognitive deficits, such as difficultly reasoning, inattentiveness, spoken grammatical errors, poor spatial sense, spotty memory loss, or dulling of emotions, or aberrant emotional behavior if the vermis is damaged. This is termed cerebellar cognitive affective syndrome (47).
Association areas in the parietal, temporal, and frontal lobes of the cerebral cortex send efferent impulses to the pons where they synapse. These areas of the cerebral cortex —parietal, frontal, and temporal lobes—are involved in integrative, complex, motivational activities. The pons also receives input from multiple areas of the brain, such as the cerebral areas associated with the limbic system and the thalamus. The temporal lobe areas associated with language, memory, and complex visual processing seem to send out impulses to the pons. The corticopontocerebellar path passes through the contralateral middle cerebellar peduncle to terminate in the cerebellar hemispheres. Schmahmann and Pandya (51) write that after the information is processed by the cerebellum, that is, “cerebellar processed,” it is returned to the cerebral hemispheres.
A second feed-forward system from the cerebral cortex to the cerebellum passes through the red nucleus, from which the central tegumental tract passes through the inferior olivary nucleus and then continues through the climbing fiber system to terminate in the cerebellar cortex (51). A return pathway has efferent fibers from the cerebellar cortex returning to the red nucleus and from the red nucleus to the thalamus. From the thalamus, via the thalamocortical tract, return impulses travel to the cerebral cortex. A further pathway to the cerebellum is from brain stem structures that contain serotonin, norepinephrine, and dopamine (51).
Electrodes in the cerebellar cortex and vermis have been used to control seizures. They have also found that the use of the electrodes has increased alertness and decreased aggression, anger, and depression (50).
The vermis (in the midline on the ventral surface medial to the cerebellar hemispheres) receives visual, auditory, vestibular, and somatosensory (head and proximal body) input (45). It has been associated with the eyes, has an influence on posture and the antigravity musculature that maintains posture associated with movements of the head, and locomotion (52). In a study of schizophrenic patients, imaging studies found pathological changes in the cerebellar vermis, but the cerebral cortex appeared normal (50). Atrophy of the vermis has been found in autistic children (50).
When all is said and done, the cerebellum likely affects nonmotor activities. How does it accomplish its ability to alter cognition and behavior? Classically, we know that the cerebellum regulates movement and its rate, force, rhythm, and accuracy. It may influence mental and cognitive functions by regulating their speed, capacity, consistency, and appropriateness (50).
Brain Stem
The brain stem is, simplistically, the brain minus the cerebrum and the cerebellum. It includes the medulla, pons, midbrain, fourth ventricle, and diencephalon (8). The “vital centers” are located in the brain stem. Some of the spinal tracts synapse, divert to terminal structures, or make horizontal crossovers (decussate) in this area. Most of the cranial nerves arise in the brain stem. The vertebral arteries, basilar artery, circle of Willis, and their tributaries feature prominently in this area. Compromise of any of these arteries may have a devastating effect on adjacent brain stem structures.
In the neonate, the brain stem has an oblique orientation and must bend to pass through the foramen magnum. It becomes more vertically oriented as the nervous system matures (2). It has some slack to allow for skeletal growth. The caudal end of the brain stem forms the beginning of the spinal cord.
Limbic System
The limbic system, or “visceral brain,” is not a specific region of the CNS but is a functional designation (53). It is an old portion of the brain. The limbic cortex, which includes the subcallosal, cingulate, and parahippocampal gyri; insula; hippocampal formation; dentate gyrus; and other structures such as the amygdaloid complex, hypothalamus, epithalamus, parts of the thalamus, and septal nuclei (part of the midbrain) all form the limbic system (8,53). The limbic system is associated with emotions and behavior. It is concerned with the basic drives for individual and species preservation (8,53). Lesions of the limbic system are thought to include schizophrenia, depression, amnesia, anxiety states, and phobias (53).
An important connection to the limbic system is the connection to the nucleus accumbens. The nucleus accumbens has been called the “pleasure” center of the brain.
The prefrontal lobotomy severs the connection between the prefrontal cortex and the limbic system. It was used to cure emotional problems but tended to leave patients passive and unmotivated.
The circuit of Papez is involved in emotions. It is formed by the fornix, a densely packed region of neurons of the hippocampal formation to the basal forebrain and brain stem. From the fornix, projections pass to the mammillary bodies of the hypothalamus and the septal nuclei. The next part of the circuit passes through the mammillothalamic tract to the anterior thalamic nuclei. From the anterior thalamic nuclei, a large projection passes to the posterior cingulate gyrus. The cingulate gyrus projects to the entorhinal or parahippocampal cortices, which sends some fibers to the hippocampus (54,55). The cerebellum, specifically the vermis and fastigial nucleus, influences the Papez circuit indirectly through its connection to various structures such as the amygdala, hippocampus, septum, PAG area, interpeduncular area, and ventral tegmental area. It is thought that the cerebellum has an inhibitory effect on the Papez circuit (56).
Spinal Cord
The spinal cord has a white matter periphery surrounding an inner gray matter core (the “gray H”). The spinal cord is covered in pia, arachnoid, and dura mater. The white matter periphery of the spinal cord is primarily composed of myelinated nerve fibers. These form the ascending and descending spinal tracts. The inner “H”-shaped portion is the region of gray matter consisting of neuron cell bodies, dendrites, and glia (34). In the center of the cord is the central canal. CSF passes through this vertically oriented canal. A blockage may cause enlargement of this canal—a condition known as syringomyelia, the classic clinical presentation of which is neurological deficits of those structures that line the canal, in particular the spinothalamic tract (pain and temperature).
The gray portion of the spinal cord is largest in the thoracic and lumbar regions of the spine because of the innervation to the extremities. The gray matter is divided into several sections. The anterior horn is the location of motor neurons. These are arrayed somatotopically. The upper body and upper extremities are located laterally; the lower extremities have a more medial location. Extensor muscles are located toward the anterior region whereas flexor muscles are located toward the posterior portion of the anterior horn (8). The dorsal horn is the entry point of the sensory fibers from the dorsal root. Processing of much of the sensory input occurs in the dorsal horn. A central section is a crossover region between the two sides and surrounds the central canal. In the thoracic and lumbar spine there is a lateral horn that is the location of the cell bodies of autonomic nerves. Rexed divided the gray matter into nine laminae with a 10th lamina surrounding the central canal (8).
The cervical cord has a large cross-section because of the considerable amount of white matter formed by all of the ascending and descending tracts that pass
through it to travel to all regions of the spinal cord. There are two enlargements of the spinal cord that are associated with innervation to the extremities. The cervical enlargement (cord levels C5-T1) is associated with the upper extremities (brachial plexus), whereas the lumbar enlargement (cord levels L1-S2) is associated with the lower extremities (lumbar and sacral plexi) (8). At the caudal end of the spinal cord is the conus medullaris. The last of the nerve roots continue on in the cauda equina, each exiting the spine at their respective levels. An extension of the pia mater and a sheathing of dura mater constitute the filum terminale and provide the caudal anchor for the spinal cord at the coccyx.
through it to travel to all regions of the spinal cord. There are two enlargements of the spinal cord that are associated with innervation to the extremities. The cervical enlargement (cord levels C5-T1) is associated with the upper extremities (brachial plexus), whereas the lumbar enlargement (cord levels L1-S2) is associated with the lower extremities (lumbar and sacral plexi) (8). At the caudal end of the spinal cord is the conus medullaris. The last of the nerve roots continue on in the cauda equina, each exiting the spine at their respective levels. An extension of the pia mater and a sheathing of dura mater constitute the filum terminale and provide the caudal anchor for the spinal cord at the coccyx.
Spinal Tracts In the evaluation of peripheral pathological manifestations of the nervous system, the clinician must have a working knowledge of the spinal tracts, which connect the peripheral nervous system to the higher centers. Below is a brief review of the major tracts and their primary functions (Fig. 9-3).
Efferent/Motor Tracts Corticospinal Tracts The corticospinal tracts are the main descending pathways and form what is called the pyramidal system. They terminate in synaptic connections onto motor neurons and sensory interneurons. The corticospinal tracts are responsible for voluntary movement and modulation of spinal motor neuron activity (38).
Lesions in the tracts or at higher levels are considered upper motor neuron lesions. The typical clinical signs of upper motor neuron lesions include hyperreflexia, muscle hypertonicity, and the presence of Babinski’s sign (38). Lesions from the anterior horn cells to the peripheral termini are considered lower motor neuron lesions—muscle flaccidity and loss of deep tendon reflexes. The neurons from the anterior horn cells to the periphery are referred to as the “final common pathway.”
Other Important Descending Tracts Vestibulospinal, reticulospinal, rubrospinal, and tectospinal tracts are among the tracts forming the extrapyramidal system. The reticular formation forms a large part of the extrapyramidal system (6). Muscle hypertonicity and involuntary movement disorders are associated with extrapyramidal lesions (6).
Ascending Afferent/Sensory Tracts
Dorsal Columns Fasciculi Gracilis and Cuneatus. The dorsal columns occupy the posteromedial region of the spinal cord. They transmit proprioception, fine touch, vibration, and two-point discrimination (57). The more laterally positioned funiculus cuneatus innervates the upper body and occupies the lateral aspect of the dorsal columns from T6 cephalically, whereas the more medial fasciculus gracilis innervates the lower body (57). The fibers ascend without interruption to the medulla oblongata and synapse in their respective nuclei (8). At this point the second-order fibers cross and form the medial lemniscus. The medial lemniscus ascends to the thalamus. From the thalamus, the impulses are projected to the somatosensory cortex.
Anterior Spinothalamic Tracts The anterior spinothalamic tracts carry “light touch” sensations to the ventral posterolateral nucleus of the thalamus (8). The fibers synapse and cross over in the anterior white commissure immediately upon entering the cord (8).
Lateral Spinothalamic Tracts The lateral spinothalamic tracts transmit pain and temperature sensations to the ventral posterolateral nucleus of the thalamus. Like the anterior spinothalamic tract, these fibers cross over immediately at their respective cord levels (8).
Spinotectal Tracts Fibers of this tract cross over immediately in the cord and ascend with the anterior spinothalamic tract. It carries nociceptive impulses to the superior colliculus and the periaqueductal gray (PAG) area (8).
Posterior Spinocerebellar/Cuneocerebellar Tracts The posterior spinocerebellar tracts carry ipsilateral unconscious proprioception to the cerebellum from the lower trunk and lower extremities; the cuneocerebellar tracts do the same for the upper torso and upper extremities (8,58). The posterior spinocerebellar tract arises in the dorsal nucleus of Clarke and ascends uncrossed to the inferior cerebellar peduncle (8,38). After the first-order neurons travel up the fasciculus cunatus and synapse in the lateral or accessory cuneate nucleus in the caudal medulla, the cuneocerebellar tract travels with the posterior spinocerebellar tract to the cerebellum (58). They are important in the coordination of the extremity muscles of posture and movement. Lesions of the spinocerebellar tracts are difficult to isolate (8).
Anterior Spinocerebellar Tract The fibers of the anterior spinocerebellar tract cross over before ascending the cord. It carries unconscious proprioception from the lower extremity (8). Impulses arrive at the cerebellum through the superior cerebellar peduncle (8). Most fibers re-cross in the cerebellum, thereby serving the ipsilateral side (58). An important function of the anterior spinocerebellar tract is to receive afferent impulses from internuncial neurons of the spinal reflex arcs, especially those of flexor reflexes (withdrawal reflex) (59). Proprioceptive and exteroceptive sensory information is important for coordinating movement and posture of the lower extremities (8).
The spinocerebellar tracts enter the cerebellar mossy fiber system and are somatotopically arranged. The mossy fiber afferents have direct and/or indirect impulses that converge from the spinal cord and brainstem, as well as the cerebral cortex. A mossy fiber can diverge impulses to nearly 8,000 Purkinje cells, the primary cells in the cerebellum (58,59). With the degree of divergence from mossy fibers and Purkinje cells, it is likely that the final termination of afferents from the mossy fibers is not just to the motor system.
Mixed and Association Tracts There are mixed and association tracts. The medial longitudinal fasciculus originates in the brainstem. The fibers arise from several nuclei. In Lissauer’s fasciculus, dorsal nerve root fibers ascend or descend up to six levels of the cord (8,60). The multilevel dispersion diffuses the sensory impulses (53). Lissauer’s zone is considered part of the structure involved in the “gate control” theory of Melzack and Wall (61). Fibers in Lissauer’s zone also merge with the spinal root of the trigeminal nerve (61). There are association tracts that are formed by the ascending or descending branches of the dorsal roots (8,44,60).
Spinal Nerves Thirty-one pairs of spinal nerves branch off of the cord. There are typically eight cervical, twelve thoracic, five lumbar, five sacral, and one coccygeal paired nerves (8).
The ventral root of the spinal nerve roots carry motor or efferent impulses. The sensory impulses enter the cord via the dorsal roots. Both somatic and visceral information travels through the dorsal root. The dorsal root ganglion contains the cell bodies of the sensory neurons.
The two roots join together to form a mixed spinal nerve that splits into ventral and dorsal rami. The dorsal ramus further divides into lateral and medial branches. The ventral ramus has a lateral branch. The spinal nerves also carry autonomic fibers to and from the sympathetic chains (see section “Functional Neuroanatomy of the Autonomic Nervous System”) (33). The somatic spinal nerves continue on to the musculature and skin of the body with motor and sensory fibers.
Somatic Peripheral Nerves The somatic peripheral system and autonomic peripheral system have intermingling fibers that confound many attempting to follow their pathways. The spinal roots carry both somatic and autonomic fibers. Some of the description of the functional anatomy of the somatic peripheral nerves is in the “Autonomic Nervous System” section because of the intermingling of fibers.
There are four major plexi formed by the ventral primary rami of the spinal nerves. They are the cervical, brachial, lumbar, and sacral plexi.
The cervical plexus is formed by the ventral rami of the C1-C4 spinal nerves (62). The muscular branches innervate many of the muscles of the anterior neck. The strap muscles of the neck, via the ansa cervicalis, arc around the internal jugular vein. The phrenic nerve is a branch of the cervical plexus and provides both motor and sensory supply to the diaphragm. Cutaneous branches supply sensory fibers to the skin and underlying tissues from the area of the clavicle over the head to the posterior scalp (55). Injury to the cervical plexus may cause disorders such as respiratory problems, neck aches, neck stiffness, cervical syndrome, or atrophy of the neck muscles (57).
The brachial plexus is formed by the C5-T1 spinal nerves and often some fibers from C4 and T2 (62). From the spinal roots, the plexus is divided into trunks, divisions, cords, and then nerves, such as the long thoracic, dorsal scapular, axillary, radial, ulnar, musculocutaneous, and median nerves, which innervate the upper torso and upper extremities (62). The long thoracic and dorsal scapular nerves innervate the interscapular muscles, which are important in scapular position. Lesions may cause Erb-Duchenne paralysis, Klumpke’s paralysis, Horner’s syndrome (57).
The lumbar plexus is formed by the ventral rami of the L1-L4 spinal nerves and some fibers from T12 (62).
The major branches of the lumbar plexus include the iliohypogastric, ilioinguinal, genitofemoral, lateral cutaneous, obturator, and femoral nerves. They give cutaneous and muscular branches to the abdominal wall down to the lower extremities (62). Lesion to the femoral nerve is called meralgia paresthetica.
The major branches of the lumbar plexus include the iliohypogastric, ilioinguinal, genitofemoral, lateral cutaneous, obturator, and femoral nerves. They give cutaneous and muscular branches to the abdominal wall down to the lower extremities (62). Lesion to the femoral nerve is called meralgia paresthetica.
The sacral plexus is formed by the spinal nerves from L4-S3. It transmits somatic, motor, sensory, and sympathetic fibers to the pelvis, lower abdomen, and lower extremities (62). The most notable branch of the sacral plexus is the sciatic nerve. Other major branches include the common peroneal, pudendal, posterior femoral cutaneous, tibial, and muscular nerves (57,62). The best known lesion of the sacral plexus is sciatica, or sciatic neuritis.
There is also a coccygeal plexus. It gives cutaneous branches to the skin in the area around the coccyx (8,62).
The thoracic spinal nerves do not form plexi but the spinal nerves send cutaneous and muscular branches along one intercostal space with some branches to adjacent intercostal spaces. This gives each intercostal space a nerve supply from two to four spinal nerves. The thoracic spinal nerves supply the skin and muscles of the chest and thoracic wall (62). A common manifestation of a thoracic nerve lesion is shingles (herpes zoster).
Afferent sensory fibers transmit impulses from receptors and nerve endings. The cell bodies of peripheral somatosensory nerves are in the dorsal root ganglion. Somatic receptors include the proprioceptors and exteroceptors. Pacinian corpuscles, muscle spindles, and Golgi tendon organs are the major proprioceptors. Free nerve endings, Meissner’s corpuscles (light touch), Pacinian corpuscles (deep pressure), Krausse end bulbs, Golgi-Mazzoni receptors, and Ruffini endings are the major exteroceptor receptors and endings (62). The area to which the sensory neuron responds is the receptive field. Areas where receptive fields are small and occur in large numbers are fields with high sensory acuity. The fingertips are an example of a highly sensitive region with a large number of small receptive fields (62).
The primary somatic efferent neurons are the motor neurons. There are two major types of motor neurons: the alpha motor neurons, which send A-alpha (Aα) and A-beta (Aβ) fibers to the striatal skeletal muscles, and the gamma motor neurons, which send fibers to the intrafusal fibers of the muscle spindles (62). The primary tracts that influence the motor neurons from higher centers are the corticospinal, vestibulospinal, rubrospinal, and reticulospinal tracts (62). A motor unit is composed of a single anterior horn cell and the muscle fibers that it innervates. In muscles from which only crude or gross movement is required, one axon innervates hundreds or thousands of muscle cells. In areas where muscles engage in fine movements, one axon innervates 10 or less muscle cells. Thigh or chest wall muscles are an example of the former whereas extraocular muscles are an example of the latter (62).
When there is damage to the motor neurons there are more motor units created upon regeneration, but this number diminishes to the original number with further tissue repair (62).
Important functions of the peripheral nerves are the reflex arcs. The simplest is the monosynaptic reflex arc. Stretch of the muscle spindle causes afferent impulses to travel up the involved peripheral afferent nerve to the dorsal root of the spinal nerve and into the spinal cord. The neuron synapses with motor neurons in the anterior horn of the spinal cord and sends impulses back to the muscle, causing contraction of the affected muscle. There are much more complex reflex arcs that involve agonist muscles and muscles of the opposite limb.
Receptors
The nervous system requires various types of receptors to sense or sample the external and internal environment. Even individual cells require “receptors,” integrins in the cell membranes.
Mechanoreceptors There are various types of mechanoreceptors. Musculoskeletal mechanoreceptors are in the muscles, myotendinous junctures, and joints. They respond to changes in muscle length, muscle tension, and joint motion. Cutaneous mechanoreceptors come in a few types depending upon function. In hairless areas, there are various types of receptors: Merkel discs (superficial skin and slow adapting, which respond to touch); Meissner corpuscles (superficial and rapid adapting, which respond to touch); Pacinian corpuscles (deep tissue and rapidly adapting, which respond to vibration); and Ruffini corpuscles (deep tissue and slow adapting, which respond to rapid indenting pressure stimuli) (63,64). Those in hair follicles are stimulated by general or light touch. Visceral mechanoreceptors respond to pressure, irritants, stretching, etc. Examples of the latter include carotid baroreceptors in the carotid sinus that respond to blood pressure changes, pulmonary stretch receptors, and stretch receptors in the walls of the stomach and bladder that signal “fullness.” Serosal mechanoreceptors are found in the organs and are stimulated by traction, compression, or distention. Mechanoreceptors in the ear are important for hearing and, through the structures of the ear, convert sound waves into nerve impulses (64).
Thermoreceptors These respond to even small changes in skin temperature. It is thought that there are thermoreceptors that detect either cold or warm
sensations. Some respond to different temperature ranges. Some respond to more moderate temperatures, whereas others respond to extreme temperatures (64).
sensations. Some respond to different temperature ranges. Some respond to more moderate temperatures, whereas others respond to extreme temperatures (64).
Nociceptors In addition to musculoskeletal mechanoreceptors, nociceptors are important to chiropractors. These are stimulated by noxious input and cause a pain response. Thermal nociceptors are stimulated by temperature extremes; mechanical nociceptors respond to mechanical forces; chemical nociceptors are activated by chemical irritants; and polymodal nociceptors are stimulated by a combination of thermal, mechanical, and chemical insults (64). Free nerve endings provide nociception in a variety of tissues.
Chemoreceptors These are sensitive to gustatory and olfactory sensations. Taste buds are modified epithelial structures on papillae on the tongue and soft palate. Each type of taste bud is sensitive to a specific taste. Olfactory chemoreceptors are specialized neurons with bulb-type endings in the olfactory epithelium. Carotid bodies are chemoreceptors in the angles of the carotid bifurcation, and aortic bodies are at the root of the aorta. These receptors are stimulated by changes in pH, oxygen tension, and carbon dioxide and will influence respiration rate and depth. There are also chemoreceptors in the gastric mucosa that are sensitive to acid levels (64).
Photoreceptors These receptors in the retina are sensitive to electromagnetic waves in the frequency of the visual spectrum (64).
Osmoreceptors These receptors are sensitive to changes in osmolality of solutions or changes in osmotic pressure. Osmoreceptors are found in the third ventricle of the brain and in the carotid artery wall (64).
Upper Motor Neuron Lesions Versus Lower Motor Neuron Lesions
When evaluating alterations to the integrity of the somatosensory nervous system that manifests in peripheral structures, the initial determination made is whether the lesion is in the peripheral nervous system or in the CNS. Clinical manifestations differ in classic ways according to the location of the lesion. The peripheral nerve in the somatomotor system begins in the anterior horn of the spinal cord. As noted, this is called the “final common pathway.” The peripheral somatosensory neurons have their cell bodies in the dorsal root ganglion.
The classic clinical signs of lesions of the spinal nerves tend to cause dermatomal sensory loss, hyporeflexia, motor weakness, and muscle atrophy. The hyporeflexia is caused by interruption of the reflex arc. The motor weakness and muscle atrophy is caused by interruption of the direct nerve supply to the muscles. The classic clinical signs of upper motor neuron lesions are hyperreflexia, clonus, and muscle atrophy.
BLOOD SUPPLY OF THE NERVOUS SYSTEM
The nervous system requires a substantial portion of the blood supply during the fetal and postnatal stages because of its rapid growth. It continues to require a major proportion of the vascular supply during adulthood as well. The high metabolic rate of the brain demands a disproportional percentage of the total oxygen and nutrient-rich blood flow required by the entire body. Seventeen percent of the oxygen-rich blood from the heart and one-fifth of the total oxygen is required by the brain for it to function. This is in spite of the fact that the brain only constitutes 2% of the body’s weight. Normal cerebral blood flow is about 50 mL/100 g brain tissue/minute and consumes about 3.3 mL of oxygen/100 g brain tissue/minute (8). The arterial supply to the brain is via the vertebral and internal carotid arteries (57).
The primary blood supply to the brain is through the two internal carotid and two vertebral arteries (8,33). These arteries join in an arterial complex called the Circle of Willis. Branches from this complex go to the brain and adjacent structures. The cerebral arteries course over the surface of the cerebrum, with branches dropping perpendicularly into its interior. This contrasts with most other organs, which have a hilus where the arteries supplying the organ enter (7).
Veins emerge from the brain and form pial plexi. These join to form the valveless deep and superficial cerebral veins, which pass through the subarachnoid space to the sinuses of the dura mater. The sinuses converge and eventually drain into the internal jugular veins (8). Of special importance is the superior sagittal sinus, which is vulnerable to head trauma. The veins of the brain stem and cerebellum tend to follow the arterial supply. The cerebellar veins drain into the veins and sinuses that also drain the cerebral hemispheres. Those of the brain stem drain into the great cerebral vein or internal cerebral veins (8).
The arterial supply to the spinal cord is from the radicular arteries and the posterior and anterior spinal branches of the vertebral arteries. The largest of the anterior radicular arteries supplies the lower thoracic and lumbar cord and is called the artery of Adamkiewicz (8,33). The thoracic spine has a relatively poor blood supply and relies on a single radicular artery (33).
The venous distribution tends to follow that of the arterial supply. The venous branches drain into the epidural venous plexus (8). The valveless venous plexus of the spine is quite fascinating. If intra-abdominal pressure is increased, venous flow from the pelvic plexus passes to the spinal plexus. Drainage from the brain may also enter the spinal plexus. Tremendous work has been done by Batson on correlating the metastasis of neoplasms of the prostate, brain, and breast to the spine. From the studies by Batson and others, it has been found that, because of the valveless nature of the spinal venous system, venous blood flow can reverse. This is the mechanism that allows circulating cancerous cells to enter the spine from remote primary sites (65,66).
VENTRICLE SYSTEM: CEREBROSPINAL FLUID FLOW
The ventricle system is composed of the two lateral ventricles; the interventricular foramen of Munro, which connects the lateral ventricles to the third ventricle; the third ventricle; the cerebral aqueduct connecting the third and fourth ventricles; the fourth ventricle; the Foramen of Magendie; and the central canal, which traverses down the spinal cord. CSF flows throughout the ventricle system and the subarachnoid space.
The lateral ventricles are located in the cerebral hemispheres. The lateral ventricles have five parts: the anterior or frontal horn, the ventricular body, the collateral trigone, the inferior horn, and the posterior horn (8). CSF passes from the two lateral ventricles via the interventricular foramen of each respective ventricle to the third ventricle. The third ventricle lies between the thalami and hypothalami. Structures such as the optic chiasma, the posterior perforating substance, and mamillary bodies lie at the floor of the third ventricle and the stalk of the pineal gland to the posterior (13). From the third ventricle, CSF passes through the singular cerebral aqueduct of Sylvius to the fourth ventricle. The fourth ventricle lies between the brainstem and medulla oblongata and the cerebellum. Many cranial nerve nuclei and tracts passing to and from the spinal cord are adjacent to the anterior aspect of the fourth ventricle. CSF in the fourth ventricle passes through openings such as the foramina of Luschka and Magendie into the subarachnoid space surrounding the brain and spinal cord and into the central canal of the spinal cord (8,44).
CSF is a clear, colorless fluid that protects, cushions, and supports the CNS (33,67). It fills the extracellular interstitial spaces and CSF cavities (67). There is about 50 mL of CSF in infants and 150 mL in adults (67). CSF is thought to be composed of an ultrafiltrate of plasma with secondary processing, which makes it effective at isolating nervous tissue (67). The choroid plexus, a blood vessel-rich network in the pia mater that projects into the ventricular cavities of the CNS, seems to be a primary origin of CSF (30). A large percentage, possibly up to 50%, is formed by the cerebral endothelium (67). The choroid plexus receives its nerve supply from vagal, glossopharyngeal, and sympathetic branches (68). CSF returns to the venous system through arachnoid villi in the superior sagittal sinuses (33,67).
INNERVATION OF THE SPINE
A very controversial issue concerns nerve supply to the intervertebral disc. In contrast, innervation to the other structures of the spine, for example, facets, ligament, and muscles, is fairly well established. Jinkins has done some research into the innervation of the intervertebral disc as has Mendel et al. (69) and Bogduk et al. (70,71). Studies have found nerve fibers in the anulus, particularly in the middle one-third of the disc. Mendel et al. (69) have found small Pacinian corpuscle-like receptors and Golgi tendon organ-like receptors in the posterolateral, upper one-third of cervical discs. Bogduk et al. (70) found branches of the sinuvertebral and vertebral nerves and ventral rami of the cervical spine innervating the discs, and have found nerve fibers and nerve endings in the anterolateral portion of the discs. Much of the nerve supply to the disc seems to be autonomic in origin, but some somatic innervation is suspected (70). Whether or not nociceptive information is obtained from these nerves has not been established (70).
The recurrent meningeal or sinuvertebral nerve innervates the structures in the area of the posterior vertebral body (60,71). It arises from the ventral root and the gray rami communicans (60,71,72) and returns to the spinal canal via the intervertebral foramen (6,74). Branches may ascend or descend one or two levels (60).
Glia
With a few exceptions, the various types of glia have been thought to be akin to foam packing around delicate structures or insulation, much as the plastic coating around wires.
Glia are a key element in the formation and operation of synapses. Astrocytes wrap themselves around synapses to monitor and regulate them. A single astrocyte can sheath more than 100,000 synapses. Plasticity in the brain is aided by the astrocytes, which secrete chemicals that induce synapse formation and regulate dendritic spine morphology, which are more stable and long term than those without astrocyte contact. The dendrites of a single neuron are sheathed by multiple
astrocytes. They also connect to capillaries to monitor and regulate the vascular supply (74).
astrocytes. They also connect to capillaries to monitor and regulate the vascular supply (74).
Oligodendrocytes wrap around certain CNS neurons to create a myelin sheath with breaks in the sheath called nodes of Ranvier; Schwann cells do the same in the peripheral nervous system. This is what we know as white matter in the nervous system. As we know, impulses that travel down myelinated neurons can travel more than 100 times faster than over unmyelinated fibers because they can skip from one node to another rather than travel through the entirety of the axons. The oligodendrocyte progenitor cells form into oligodendrocytes. They are found not only in the immature CNS but also in the adult. They have been found to have voltage-gated ion channels and neurotransmitter receptors (74).
Learning new skills, from physical skills in sports to learning languages, is best done while myelination is occurring. The younger you are when you learn a skill, the greater is the potential to be more skillful than someone who tries to learn later in life. World-class athletes begin as children. A child who learns a language will have less of an accent when speaking than someone who learns it later in life. However, someone who begins learning a skill or complex intellectual endeavor still stimulates myelination, no matter their age (75).
Like astrocytes, Schwann cells can influence synapses. They guide growth cones to the muscles and cause the development of, maintains, and adheres to the synapses at the neuromuscular junction (74).
Astrocytes are part of the blood-brain barrier and are involved in ion homeostasis. Microcytes are part of the CNS’s innate immune system (75) and have macrocytic origin. Microcytes can respond by macrocytic reaction or secretions of neutralizing cytotoxins to inflammatory, immunologic, ischemic, or traumatic insults (74).
It has been found that glia are involved in synaptic transmission and have neurotransmitter receptors. They can receive synaptic transmissions and release neurotransmitters to neighboring glia and neurons. They have also been found to direct synaptic connection to neurons. They do not have axons nor generate action potentials.
Astrocytes create signal waves with intracellular calcium between astrocytes (76). Astrocytes have also been found to have bidirectional communications with neurons (77). They can also monitor axon transmission and, if axonal traffic is increasing, they can chemically stimulate the oligodendrocytes to form more myelin (75).
Glia are sensitive to environmental factors. From the late fetal period to adolescence, when myelination is occurring, tobacco smoke can reduce the formation of myelin (75).
FUNCTIONAL NEUROANATOMY OF THE AUTONOMIC NERVOUS SYSTEM
The ANS is probably the most complex and least understood system in the body. That many names have been applied to the ANS attests to its anatomic and physiologic complexity and its uniqueness and importance to health and function. It has been called the vegetative, involuntary, visceral, plexiform, and major sympathetic nervous system (SNS), among many other names. It is composed of a strikingly complex configuration of chains, cords, ganglia, nuclei, plexi, nerves, and filaments that do not lend themselves to effortless understanding. The cerebrospinal system seems almost map-like in comparison. The “autonomic nerve system” was formally disseminated as a distinct neural feature in 1905 by Cambridge University physiologists J. N. Langley and W. H. Gaskell after they observed that specialized receptor sites on end-organs and ganglia had to be chemically stimulated by a number of specific substances of different classes for autonomic effects to take place (78).
The ANS has been described as a system that “makes nothing but is responsible for everything.” Irwin Korr stated that stimulation of the ANS does not introduce new qualities, but rather it modifies by increasing or decreasing, accelerating or retarding, or stimulating or inhibiting the physiological properties and actions of the target tissue. The modified target tissue action or property then has its own specific effect on total body physiology (79). The ANS influences all unconscious operations, leaving little that it does not control, except the volitional movements of striatal muscles, in which it also has an effect: it is indispensable to the function of striatal muscle through neural and cardiovascular tone.
The chiropractic clinical effect and so-called “philosophical” position on health has been historically and popularly stated as coming “from above down, inside out,” or “from within.” Disease is an abnormal physiological process based on neural dysfunction; chiropractors do not cure disease, but they remove the “neural interferences,” thereby allowing the “innate intelligence” of the body to be more fully expressed. The ANS seems to provide the primary neurological pathway for health, homeostasis, and “innate intelligence” to be expressed. This section of the chapter will explore both the anatomic and functional characteristics of the ANS.
Derivation of the ANS
The human nervous system is a derivative of both the ectodermal and mesodermal layers of the embryo. Early cellular and structural differentiations include the cells of and form of the notochord at about 10 days of gestation. This signals the formation of the neural tube from
the ectodermal layer, which will appear around the third week. The neural tube will form the CNS. The peripheral and enteric nervous system will be made up of neurons and glial cells that emerge from lines of neural crest cells located along the lateral neural plate margins. They are stimulated by adjoining non-neural ectodermal cells. Neural cells are directed by a largely conjectural mechanism—signal downstream transcription—to various sites around the neural tube. The lateral portions of the neural tube become the brain and spinal cord. The notochord becomes the anterior portion of the spinal column and somites. Some of the neural crest cells will form smooth muscles and pigment cells. Interestingly, in some organisms, the pigment cells or chromatophores subsequently are directed by the ANS to change their color in response to the environmental conditions. Both extracellular—from other embryonic organs— and intracellular signaling molecules, as well as a host of other neurotrophic factors, are needed to direct the ultimate course to the periphery and fate of the crest cells. The development of specific types of neurons and their connections are matched to appropriate receptors on corresponding target tissue by careful orchestration of these molecules. Development of the ANS continues with the formation of parasympathetic and sympathetic nerve tissues, which are established in various central nerve system centers and tracts and peripheral nuclei, projections, and ganglia to ultimately release acetylcholine and epinephrine classes of transmitters at neuroneuronal and neurotarget interfaces (80).
the ectodermal layer, which will appear around the third week. The neural tube will form the CNS. The peripheral and enteric nervous system will be made up of neurons and glial cells that emerge from lines of neural crest cells located along the lateral neural plate margins. They are stimulated by adjoining non-neural ectodermal cells. Neural cells are directed by a largely conjectural mechanism—signal downstream transcription—to various sites around the neural tube. The lateral portions of the neural tube become the brain and spinal cord. The notochord becomes the anterior portion of the spinal column and somites. Some of the neural crest cells will form smooth muscles and pigment cells. Interestingly, in some organisms, the pigment cells or chromatophores subsequently are directed by the ANS to change their color in response to the environmental conditions. Both extracellular—from other embryonic organs— and intracellular signaling molecules, as well as a host of other neurotrophic factors, are needed to direct the ultimate course to the periphery and fate of the crest cells. The development of specific types of neurons and their connections are matched to appropriate receptors on corresponding target tissue by careful orchestration of these molecules. Development of the ANS continues with the formation of parasympathetic and sympathetic nerve tissues, which are established in various central nerve system centers and tracts and peripheral nuclei, projections, and ganglia to ultimately release acetylcholine and epinephrine classes of transmitters at neuroneuronal and neurotarget interfaces (80).
Phylogeny
It has been postulated that the human ANS has undergone three phylogenic phases of development that, like the brain, are evolutionary progressions. Some of these developmental phases have taken place at the same time that the central and peripheral nervous systems are thought to have evolved, but some developed independent of the phases. The most primitive form of nerve system is seen in the jellyfish, in which it acts as a nerve network. Nerve fibers are distributed uniformly throughout the jellyfish’s cylindrical body. As later species evolved, the neural fibers condensed and clustered toward a midline, with some neural extensions remaining in the periphery. The central neural fibers aggregate into two or more longitudinal nerve cords along the dorsal and ventral surfaces. The cords unite at the head or anterior and by ladder-like transverse commissures between the remaining length of the two cords. Nerve fibers further cluster along the longitudinal axis and demonstrate clearly an evolutionary movement toward the development of central neuronal reflex control. At the intersections of the longitudinal and transverse commissures, neural clusters form primitive ganglia. These ganglia develop more numerous and densely at the head, presumably because organisms project themselves unidirectionally and encounter and respond to new environmental conditions cephalically. The dorsal surface neural cord eventually dominates and continues the tendency towards a single, central reflex control of neural activity. Then, interestingly, the dominance of the dorsal and ventral cords influence, in essence, switches in response to the organism locating more of its body contents from the posterior to the anterior. The ventral primary division in mammals will be required to expand functions, whereas the dorsal roots will have limited functions. The peripheral nerve cells in earlier or more primitive organisms transmit impulses freely along the nerve in either direction. Later, the nerve cell impulse traffic becomes increasingly unidirectional, either transmitting impulses toward or away from the central cord. The nerve cells that remain in the clusters within the central cord may develop into spinal cord internuncial association cells, which then connect to other neurons ipsilaterally and/or bilaterally at the same levels and/or at levels above or below. The enteric nerve system in humans, with its extensive intramural plexi, is thought to be representative of the “nerve network” found in the lower species, such as the jellyfish (81).
The first phase of ANS development produces the vagus nerve system. It is formed by unmyelinated nerve fibers. It generally serves to augment digestion in the central cavity and to respond to an external threat by depressing metabolism and immobilizing the organism so as to not incite a reflex chase or strike response (“playing ‘possum”). The SNS forms in the second stage of development to provide the opposite response. It inhibits the action of the visceral vagus nerve, and its interofective/internal actions increase metabolism and mobilize the organism. This thereby produces the “fight or flight” response. The third phylogenic development of the ANS takes place only in mammals. During this phase, the vagus nerve is myelinated. It develops the ability to regulate some aspects of cardiac function by mingling with the sympathetic nerve that supplies the heart. This addition is seen as a means to fine-tune the “fight or flight” response of the sympathetic system through suppression of certain aspects of the sympathetic response. Physiological restraints offer possibilities for more safety within the social group and more social organization. Mammals, which have fewer offspring and require longer gestations than other vertebrates, have greater mating opportunities and success, with more mate bonding interactions and more child-rearing success. Increased myelinization occurs in the cranial nerves as well. The result is an expansion and refinement of facial and voice expressivity. Thus, the vagal changes modify the individual’s self-centered “fight or flight” response in favor of group directives
and goals, which potentially adds a collective adaptive advantage to a group. This adaptation is the basis for nonreflexive social behaviors. The developments tie into musculoskeletal activities that range from group flight or stand to individual actions, such as assuming postures that are more conducive for reproduction and nurturing (82,83).
and goals, which potentially adds a collective adaptive advantage to a group. This adaptation is the basis for nonreflexive social behaviors. The developments tie into musculoskeletal activities that range from group flight or stand to individual actions, such as assuming postures that are more conducive for reproduction and nurturing (82,83).
Anatomy
Like the cerebrospinal nervous system (CSNS), the ANS has central and peripheral processes with both efferent and afferent fibers going to effectors and receptor terminals. The central processes of both systems are located within the spinal cord and brain; the peripheral processes are those structures that are external to the brain and spinal cord. A feature that distinguishes the ANS from the CSNS is the additional level of peripheral integration and complexity at the peripheral ganglia. The ANS’s equivalent of the CSNS’s “final common pathway” originates from these external peripheral ganglia, the neural extensions which further to the periphery are called “postganglionic nerves” (Fig. 9-4). This suggests that many ANS responses have more local control. The synapses of these ANS pathways are more open to influence of a variety of circulating or locally produced neurotransmitters and neuromodulators.
Though both the CSNS and ANS have peripheral components involving afferent and efferent nerve fibers, communication between the autonomic central nuclei and autonomic targets takes place by either sympathetic or parasympathetic pathways. These pathways have their own unique designs, with distinct central origins, peripheral distributions, and responses to stimuli (84). Most viscera have dual enervation from both the SNS and parasympathetic nervous system (PSNS). These complex systems of dual enervation are much like a dual key system of a safe deposit box—one key is held by an avid spender, the other by a saver—which allows careful control of the manner in which the tissues will function.
The chiropractic tradition has portrayed the relationship between the structures within the central and peripheral nerve systems by employing a simple “safety pin” cycle diagram. Very elaborate versions of this cycle were developed by early chiropractic pioneers. A slightly more elaborate version of the “safety pin” cycle will serve as the foundation for this section’s description of the regions and functions of the ANS in the brain, spinal cord, proximal and distal nerve pathways, and organ/tissue plexi. ANS anatomy and functions can be conceptualized, at least in part, as arising from and coursing within the following generalized schema. The slightly elaborated safety pin cycle diagram consists of two links. The vertical, or central, connections include input from descending tracts that originate in the brain and the internuncial connections from adjacent vertical levels of the spinal cord. The horizontal, or peripheral, pathway connections include all input from the peripheral sensory receptors (both autonomic and somatic) and adjacent horizontal spinal cord internuncial connections.
Central Structures of the ANS
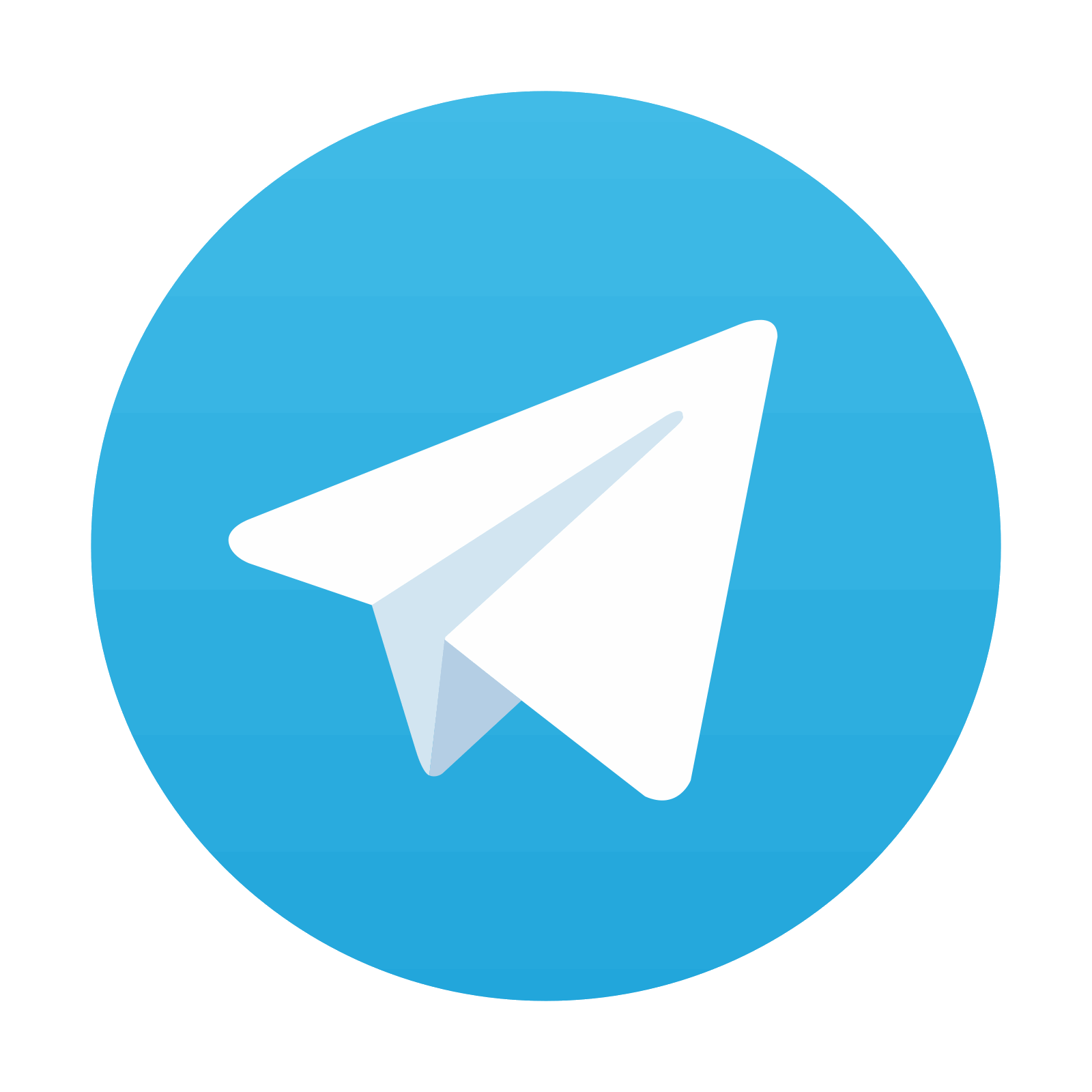
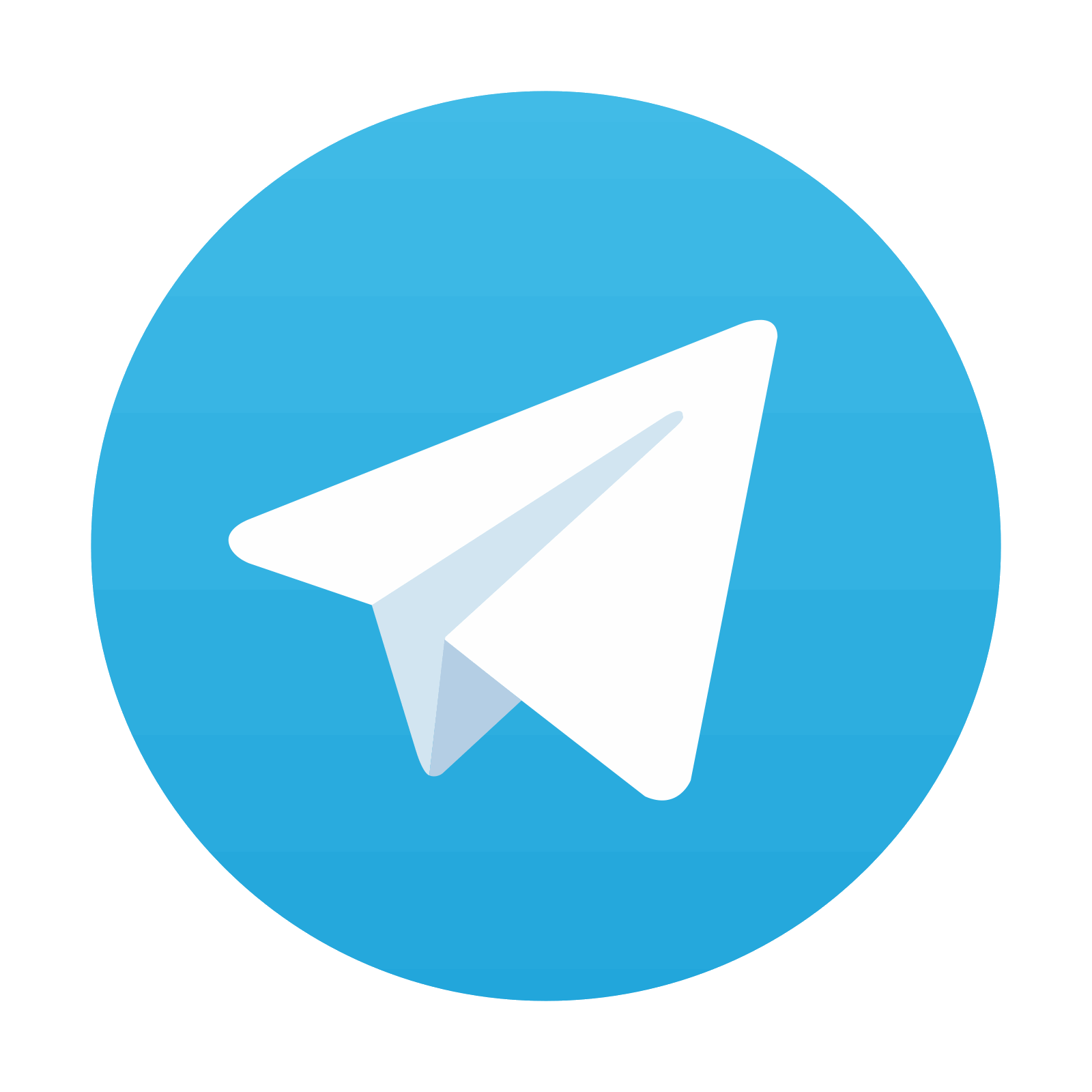
Stay updated, free articles. Join our Telegram channel
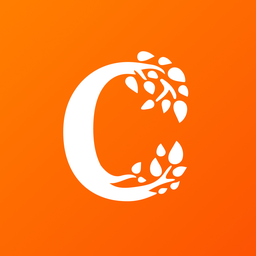
Full access? Get Clinical Tree
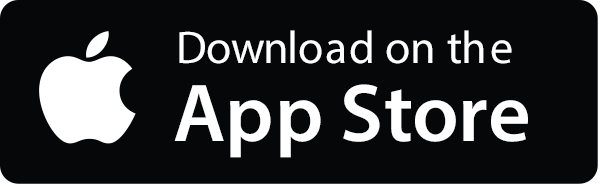
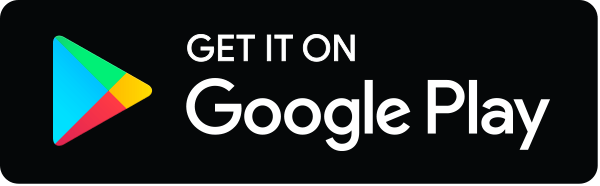
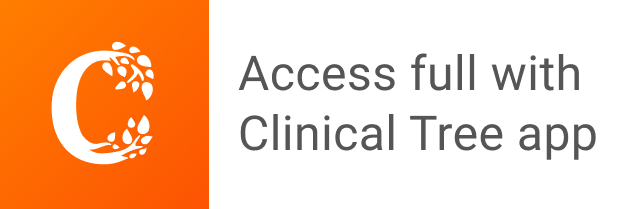