Abstract
Our genetic information, sometimes described as the “Book of Life,” can be envisaged as a compilation containing two “sets” of information, with one originated from the female and the other from the male. Each set includes 23 “volumes” (chromosomes), and each of these chromosomes contains many “chapters and pages” (clusters of genes). Each gene encodes for a specific message to the cell cytoplasm, where ribosomes translate the codes to specific proteins. The genetic code is made up of combinations of three out of four possible essential nucleotides (A, T, G, and C). These nucleotides are the chemical molecules, components of deoxyribonucleic acid (DNA), creating the genetic information that can be further transcripted and translated by different cell types of machinery to produce the essential cellular proteins. A trinucleotide code (three basic nucleotides), called a codon, encodes a specific amino acid, a basic brick of protein structure.
Genes and Chromosomes
Our genetic information, sometimes described as the “Book of Life,” can be envisaged as a compilation containing two “sets” of information, with one originated from the female and the other from the male. Each set includes 23 “volumes” (chromosomes), and each of these chromosomes contains many “chapters and pages” (clusters of genes). Each gene encodes for a specific message to the cell cytoplasm, where ribosomes translate the codes to specific proteins. The genetic code is made up of combinations of three out of four possible essential nucleotides (A, T, G, and C). These nucleotides are the chemical molecules, components of deoxyribonucleic acid (DNA), creating the genetic information that can be further transcripted and translated by different cell types of machinery to produce the essential cellular proteins. A trinucleotide code (three basic nucleotides), called a codon, encodes a specific amino acid, a basic brick of protein structure. Also, other DNA motifs signal the ribosomes to start or stop producing proteins.
In human, each somatic cell contains 23 pairs of chromosomes (46 in total, 44 autosomes and 2 sex chromosomes, marked as 46,XX for female and 46,XY for male). However, in gametes (oocyte and sperm cell), only one set of chromosomes is present (23 chromosomes). Thus, we describe the chromosome constitution in somatic cells as “diploid,” meaning two sets of 23 chromosomes, and as “haploid” in gametes when only one set remains. During the formation of the oocyte or sperm, chromosome pairs exchange homologous regions and separate, therefore only one copy of chromosomes is found in each gamete. Errors occurring in chromosomal pairs and chromatid segregation, during the first and second meiosis, result in very common aberrations in a fertilized cell such as an extra chromosome – trisomy, or loss of a chromosome – monosomy.
The chromosomes are organized in the nucleus of all cells and encode for ~20 000 genes. A small number of genes are included out of the nucleus, in mitochondria, the energy centers of the cell. The function of more than 95% of the DNA is still unclear, while only ~1% of DNA “carries a message” and is often called “coding DNA” (genes coding region). Even in those active regions, only exons are transcribed to mRNA, which exits the nucleus and is finally translated into proteins. Contrary, the introns flanking the exons remain in the nucleus and do not express. Although all cells in individuals have an identical genetic constitution, the genes in each organ and tissue are differently active and expressed. Regulation of gene expression is highly controlled by transcription factors, translation regulation, and epigenetic mechanisms.
Types of Mutations
Recessive mutations. All individuals carry several mutated genes on their chromosomes, which pass on to their offspring. However, usually, family members do not present any clinical symptoms as a result of these changes. “Silence” of the mutation occurs due to the existence of two gene copies, a mutated one and a normal one. When the mutated gene fails to express its coding protein, the other healthy copy gene frequently leads to normal development and function. When both copies of a gene are mutated, there may be no protein production at all or an abnormal production that probably results in a genetic disease.
Dominant mutations.
Expression of a mutation that appears only in one of the gene copies hints a dominant pattern of inheritance due to overriding the typical gene sequence or “dominance.” Dominant mutation can be inherited from one of the parents; however, in many cases, a dominant mutation occurs during gametogenesis, or when the fertilized oocyte starts the first divisions to produce new cells. These mutations are referred to as de novo dominant mutations. In such a case, the affected offspring will be the first individual in the family affected by this condition. There are some genes with a greater tendency for these de novo events as in cases of dystrophin, neurofibromatosis, tuberous sclerosis complex genes, etc. This de novo mutation occurs probably due to the gene size, genetic structure, or specific hot spots with a predisposition for molecular aberrations.
There are three major types of genetic disorder, which are classified according to the origin of the genetic aberration: monogenic disorders (usually due to a point mutation in a single gene), chromosomal disorders (structural changes in the chromosomes or the gain or loss of an entire chromosome), and multifactorial disorders (due to the interaction of several genes and influence of environmental factors such as diet, chemical exposure, and lifestyle on the expression of genes). Some persons have a higher risk of developing illness than others, due to a specific mutation considered to be “genetically predisposed.” Several examples for predisposition include the mutations in mismatch repair genes as in cases such as BRCA1, BRCA2, MSH2, MSH6, etc. In mutation carriers of these genes, the risk for cancer is dramatically increased and it tends to appear at an early age and sometimes in several organs.
Some genetic disorders occur more frequently in some populations. For example, cystic fibrosis and hemochromatosis are seen more often in people whose ancestors were European. At the same time, thalassemia is found more frequently in people whose ancestors came from Southern Europe, the Indian subcontinent, the Middle East, Africa and Asian countries.
Monogenic Mutations
The majority of point mutations take place in the coding region and are derived from substitutions of one letter with another in a codon that can result in the production of a different amino acid or in a stop codon that terminates protein synthesis. Other mutations originated from the deletion or insertion of single or several nucleotides that usually leads to a shift in the reading frame of genetic information and termination of translation. Splicing mutations that usually result from the destruction of consensus sequence flanking the exons are also responsible for severe genetic conditions. The genetic expression of point mutations can vary from mild to severe condition and depends on transcription and translation impairment. Examples of disorders that occur due to monogenic mutations include cystic fibrosis, thalassemia, Tay–Sachs disease, familial dysautonomia, BRCA mutation, Gaucher and Bloom diseases, and many others.
Another type of monogenic mutation refers to insertion or deletion of hundreds or thousands of nucleotides, as in cases of hemophilia, Duchenne and Becker types of muscular dystrophy, and neurofibromatosis.
In general, a mutation is usually inherited from one of the parents, but it can also occur de novo during gametogenesis or early embryo development.
An additional type of monogenic mutation is the expansion of trinucleotide repeats in specific genes. If the number of repeats increases beyond a critical point, the gene can become “unstable” and may develop further repeats. For example, in fragile X syndrome, CGG repeats are localized in the promoter region of the gene and exceed in repeat numbers over 200 leads to complete inhibition of transcription due to the gene hypermethylation. Other mechanisms characterize different severe disorders, also originated from trinucleotide expansions, as in cases of Huntington’s disease and myotonic dystrophy.
Mutations in genes on the X chromosome.
Mutations in genes carried on the X chromosome are different in females and males. Females have two copies of the X chromosome, while males have only one X chromosome. Unlike chromosome Y, chromosome X is considered to be “gene-rich,” with many genes encoding proteins significant for growth and development. These “gene-rich” genes include genes for the synthesis of muscles’ structural protein dystrophin, and several proteins that control blood clotting. When these particular X chromosome genes are mutated, specific genetic disorders, especially in males, result such as Duchenne muscular dystrophy, hemophilia, fragile X syndrome, etc.
Shortly after conception, one of the X chromosomes in each female cell is randomly “switched off” so that (except a few chromosomal regions that are not included in inactivation) males and females both have only one active X chromosome in each cell. Females, who have a mutated gene on one X chromosome, are “carriers” of the gene mutation. The mechanism by which the X chromosome is “switched off” is random. Thus, some cells will have the X chromosome coding for the correct product, while other cells will produce the abnormal product due to the mutation; the correct copy usually protects carrier females from any effects of faulty genes with a recessive inheritance on the X chromosome. Males have no other X chromosome to provide “backup” and will usually be affected due to the mutated X chromosome gene expression, and a disorder may be seen at birth or later in life. Therefore, for disorders caused by recessive mutations on the X chromosome, males in the family are often affected, whereas the females can be unaffected carriers of the mutation.
X Chromosome Inactivation
Inactivation of one copy of the X chromosome in female cells safeguards that both males and females have the same number of X chromosome genes instructing the body to grow, develop, and function. This system of “switching off” of most of the X chromosomes is seen in all mammals and is often called lyonization, named after Mary Lyon, who first clearly described the system in 1962. In humans, it occurs very early in embryonic development. One of the X chromosomes turns out to be very shortened and condensed such that most of its genes cannot be “read” by the cell; the dark body in the cell, called a Barr body, is the inactivated X chromosome. X chromosome inactivation only occurs in the somatic cells of the early embryo (inner cell mass at blastocyst stage), since both X chromosomes need to be active in the oocytes for their normal development. This system of inactivation is usually random, i.e., some cells will have the maternal X chromosome switched off, while other cells will have the paternal X chromosome inactivated. X chromosome inactivation affects most of the genes located on the X chromosome, but not all. Particular genes on the X chromosome have their “partner”; they are active on both chromosomes, such as the gene called ZFK that codes for a protein that is possibly involved in the production of both oocytes and sperm cells.
Mitochondrial DNA
DNA also exists in mitochondria. There are over 80 different genes required to produce the components of the mitochondrial respiratory chain. Defective mitochondrial DNA relates to mitochondrial disorders. The expression and severity of the mitochondrial disorder depend on the ratio between mitochondria with mutation and those free of the mutation.
Chromosomal Structural Rearrangement
Aberrations in chromosome structure may result from the addition or loss of chromosomal material and may occur in many ways, but most commonly by translocation of a part of a chromosome, microdeletion, microduplication, inversion, disomia, or mosaicism.
Translocations.
Sometimes, a piece of one autosome or sex chromosome is broken off and attaches to a different autosome or sex chromosome in a process called translocation, occurring in around 1:1000 people. There are two common types of translocation: reciprocal – two chromosomes exchange chromosomal segments in the regions where breakage occurs, and Robertsonian translocation – two acrocentric chromosomes are abnormally sticking one with the other and both are losing their p arms. If all the genetic information is present, but simply rearranged, such a translocation is described as “balanced.” If translocation has an uneven distribution of chromosome material, such a translocation is described as “unbalanced.” An unbalanced translocation, involving large chromosomal fragments, is usually lethal and aborted during early pregnancy. Unbalanced translocations of smaller DNA regions may result in a viable offspring with possible physical or mental developmental disorders, due to a gain or loss of chromosome material. If a woman is a carrier of a translocation between an autosome and the X chromosome, there is a high chance that she will be infertile.
A Robertsonian translocation (named after an American cytogeneticist) is a particular type of rearrangement mostly involving chromosomes 13, 14, 15, 21, and 22. These structural rearrangements are found in about 1:1000 of the average population. The most common of these (~33% of all Robertsonian translocations) are between chromosomes 13 and 14. Couples, where one partner is the carrier of a Robertsonian translocation, can experience repeated miscarriage and male infertility [1–2].
Deletions.
A small part of a chromosome may be lost or deleted during the formation of gametes. If the missing material contains essential information for the body’s development and function, a disorder will result. Large deletions are usually lethal. A child with cri du chat syndrome has a small part of the short (p) arm of chromosome 5 deleted, causing a range of disabilities, including a characteristically high-pitched mewing cry in infancy. Deletions are one of the genetic variants causing male infertility [3].
Duplications.
A small part of a chromosome may be gained or duplicated along its length. This results in changes to the number of genes present and may result in health, development, or growth problems.
Inversions and rings.
Sometimes the chromosomes twist around themselves, become inverted, or joined at the ends to form a ring instead of the usual rod shape. During the formation of these structures, some genetic material may be lost. Inversions and rings may cause problems in physical and/or mental development due to an imbalance of chromosomal material, as well as genetic variants causing male infertility [4].
Uniparental disomy.
Uniparental disomy occurs when both members of a pair of chromosomes come from one parent. This phenomenon generally does not cause health problems, except for cases where it is necessary to have one chromosome from each parent due to differential chromosomal imprinting.
Mosaicism.
Most people have the same chromosome pattern in all cells in their bodies. People who are chromosomally “normal” would thus have either 46,XX or 46,XY in all their cells. Just as mosaic tiles on a floor have a mixture of patterns, some individuals present a chromosomal mosaic and have a mixture of chromosomal patterns in different cells – usually a mixture of cells with the correct number or structure of chromosomes, together with cells carrying a chromosomal abnormality. The proportions of chromosomally abnormal and normal cells can be quite variable and may also vary between different body tissues. For illustration, people with mosaic for trisomy 21 may have the chromosomal abnormality in 60% of cells in their skin versus only 5% of their blood cells. Some mosaics have a mixture of cells with different abnormal patterns. It is not always known for sure whether an individual is a mosaic. Even in cases where mosaicism is established, the pattern in different parts of the body is not always known, rendering it challenging to predict how severely affected a person may be. An embryo classified as mosaic has a chance of 35% to produce a viable pregnancy, whereas a healthy embryo has a chance of 65% and an abnormal embryo has a chance of less than 4%.
Genetic Testing of Male Infertility
Approximately 2300 genes are involved in the process of spermatogenesis. Spermatogenesis checkpoints are controlled by a multitude of genes and signaling pathways that regulate mitosis, meiosis, and sperm transport. Synaptic defects during meiotic prophase in the male almost always result in spermatocyte death, either at the pachytene stage or at first meiotic metaphase. By contrast, females retain fertility in the face of many mutations that cause complete meiotic arrest and sterility in males. The less stringent pachytene checkpoint mechanisms in the female are likely to underlie the male versus female differences in sex chromosome sensitivity during meiosis. Meiotic sex chromosome inactivation occurs only in males, which explains the difference in male versus female sensitivity to the presence of unsynapsed chromatin. It has been hypothesized that failure to inactivate the sex chromosomes is the leading cause of asynapsis-related male sterility [5–6]. Male infertility accounts for 15–30% of infertility cases, most of them with known genetic factors [7]. Common genetic abnormalities include chromosomal or gene defects and epigenetic alterations. Infertile men may appear with phenotypic abnormalities or with sperm abnormalities. Phenotypic abnormalities include Klinefelter syndrome, congenital absence of vas deferens, Kallmann syndrome, cryptorchidism, hypospadias, ambiguous genitalia, and androgen insensitivity syndrome. Sperm DNA damage has been implicated in both primary infertility and recurrent spontaneous abortions following natural and assisted conception. Sperm DNA damage can be transmitted to offspring and may increase the risk of intergenerational infertility or other serious health problems, such as congenital malformations and childhood cancer. Genetic screening includes cytogenetic analysis to detect numerical and structural chromosomal rearrangements, analysis of single-gene mutations, and assessment of nonspecific DNA damage.
A variety of techniques are necessary for such genetic analyses: gene copy number variations and single-gene mutations/polymorphisms are detected by Sanger DNA sequencing, deletions/microdeletions are detected by polymerase chain reaction (PCR), and cytogenetic tests such as karyotyping detect chromosome aneuploidies. Cytogenetic analysis is the most common diagnostic genetic test used to evaluate men with severe oligozoospermia and azoospermia [8]. The incidence of cytogenetic abnormalities in infertile males ranges from 2.1% to 15.5% [9–10].
Genetic Testing of Female Infertility
In females, genetics plays a role in infertility; these effects are mostly polygenic, rendering it challenging to define a single genetic cause. The difference between spermatogenesis and oogenesis begins after pachytene: male gametes proceed quickly through the rest of meiosis, while oocytes arrest in a late stage of prophase for weeks to months, if not decades. For women with unexplained infertility issues, genetic tests such as karyotyping or DNA sequencing of selected genes are performed. The two most common female factor conditions, ovulatory dysfunction (25%) and endometriosis (15%), have a familial predisposition, suggesting a genetic basis [11]. Also, sex chromosome alterations [12] and several single-gene mutations have been shown to impact female fertility [13], causing conditions such as hypogonadotropic hypogonadism, premature ovarian insufficiency, endometriosis, and polycystic ovary syndrome. Most aneuploidies are due to errors in maternal meiosis and occur in approximately 65% of oocytes when maternal age is 36 and over, with rates increasing with maternal age [14].
There are many ways in which chromosome dynamics can be disturbed in oogenesis and, consequently, there are many routes to human aneuploidy. The impact of maternal age points to multiple mechanisms by which aging affects chromosome segregation. The subsequent loss of sister chromatid cohesion from chromosome arms at anaphase I and from sister centromeres at anaphase II is essential to orchestrate the complex chromosome segregation events necessary to produce haploid gametes. Failure to establish connections between homologs is one of the oldest postulated mechanisms of human aneuploidy [15] and studies of human trisomies suggest that recombination failure is, indeed, an essential mechanism of human nondisjunction. It has also been suggested that the premature separation of sister centromeres is a major mechanism of human aneuploidy [16]. Because cohesion is established during the premeiotic S phase in the fetal ovary, but chromosome segregation occurs years later in the adult, the idea that degradation of cohesion occurs during the protracted meiotic arrest is the basis of the effect of human maternal age on chromosome segregation abnormalities. Cohesins observed during fetal development are necessary and sufficient to mediate cohesion in the adult fully mature oocyte [17–19]. Meiotic recombination occurs in the fetal ovary and failure to recombine and/or suboptimally located crossovers are prominent contributors to human trisomy. Incidence of chromosome misalignment on the first meiotic spindle and disruptions of the anaphase-promoting complex (regulation of the progression from metaphase to anaphase) result in an increased incidence of aneuploidy [20].
Cytogenetic Analysis and Reproduction
Cytogenetic analysis, e.g., karyotyping, is the most commonly used diagnostic genetic test for the evaluation of men with severe oligozoospermia and azoospermia.
Klinefelter syndrome.
Klinefelter syndrome is the most frequent cytogenetic/sex chromosomal/numerical anomaly (aneuploidy) detected in infertile men and leads to nonobstructive azoospermia. Men with Klinefelter syndrome have a 47,XXY chromosomal complement in all cells or are “mosaic” [21–23]. The karyotype arises spontaneously when paired X chromosomes fail to disjoin in the first or second phase of meiosis during oogenesis or when XY chromosomes fail to segregate during spermatogenesis. The presence of two X chromosomes in Klinefelter syndrome leads to seminiferous tubule dysgenesis in approximately one-third of all cases. The failed inactivation of the X chromosome is the most plausible reason for impaired spermatogenesis in these patients. The frequency of Klinefelter syndrome is 0.2% of male newborns and 11% of azoospermic men [24]. The adult Klinefelter syndrome testis shows extensive fibrosis and hyalinization of the seminiferous tubules [25]. However, foci of spermatogenesis can be present in these patients, allowing them to become a genetic father after testicular sperm extraction (TESE) and subsequent intracytoplasmic sperm injection. In its non-mosaic form, Klinefelter syndrome is associated with elevated serum follicle-stimulating hormone (FSH) and luteinizing hormone (LH) levels, as well as low testosterone levels. The sterility in Klinefelter syndrome is due to the high prevalence of azoospermia, seen in 92% of Klinefelter syndrome men capable of providing a semen sample, with the remainder having a median of 0.1 million sperm cells/ml. It has also been reported that there is an advanced decline in spermatogenesis by age in men with Klinefelter syndrome; hence early sperm retrieval or sperm banking (if possible) is advocated in such cases [26–27]. Controversial data show that collecting spermatozoa by TESE at early adolescence does not appear to result in higher sperm retrieval efficiency compared with TESE at adult age [28]. Moreover, histological studies have shown that only small numbers of spermatogonia are present in the testicular biopsies from adolescent boys [29] and germ cells are lost at a very young age, even before the initiation of the fibrotic process. However, focal areas of spermatogenesis may be present within the testis of azoospermic men with Klinefelter syndrome, with sperm successfully extracted in approximately 40–50% of cases after TESE [30]. In most men with nonobstructive azoospermia, clinical parameters do not predict success in sperm retrieval. The majority of offspring born from 47,XXY fathers are genetically normal, although chromosomally abnormal fetuses have also been reported [31–32]. Fluorescence in situ hybridization analysis has demonstrated that the frequency of sex chromosome aneuploidy is 1.5–7% in sperm from Klinefelter mosaic patients [21], and ~45% in the sperm of men who appear to have a non-mosaic 47,XXY karyotype [23; 33].
Men with Klinefelter syndrome, with serum testosterone levels of less than 250 ng/dl, treated with aromatase inhibitors, clomiphene citrate, or human chorionic gonadotropin before micro-TESE responded to treatment, leading to an elevation of testosterone to 250 ng/dl or higher, and higher sperm retrieval rates compared with men in whom post-treatment testosterone levels were under 250 ng/dl (77% vs. 55%) [31].
The 46,XX male syndrome, also known as De la Chapelle syndrome, is a rare aneuploidy, with approximately 90% of these males showing translocation of a sex-determining region on the Y (SRY) chromosome to the X chromosome or an autosome [34–35]. SRY is a gene responsible for the testicular determination and is essential for initiating testis development and differentiation of the bipotential gonad into Sertoli cells, which then support differentiation and development of the male germline. When the paternal X with the SRY gene combines with a normal X from the mother during fertilization, the result is an XX male. Although 46,XX males exhibit normal internal and external male genitalia, they lack the major spermatogenic regions (basically on the Y chromosome) and are azoospermic [36].
Jacob’s syndrome.
The genetic cause of this syndrome, 47,XYY, is paternal nondisjunction during meiosis, resulting in YY sperm [37]. These patients exhibit tall stature, and 1–2% of them show aggressive behavioral characteristics. Most are azoospermic or severely oligozoospermic and testis biopsy findings range from Sertoli cells only to maturation arrest patterns. Unlike Klinefelter syndrome, serum testosterone levels are normal.
Triple X syndrome.
Trisomy X (47,XXX) is a genetic disorder that affects about 1 in 1000 females and most commonly occurs as a result of nondisjunction during meiosis [38]. Many females with triple X syndrome experience no or only mild symptoms; it is therefore estimated that only 10% of individuals with trisomy X are actually diagnosed. Most females with triple X syndrome experience regular sexual development, presenting with late menarche and menstrual irregularities in some cases, and can become pregnant. A taller than average stature is the most typical physical feature. Symptoms in females with triple X syndrome may include an increased risk of delayed development of motor skills, such as sitting up and walking, as well as speech and language skills, dyslexia, hypotonia, behavioral problems, such as attention-deficit/hyperactivity disorder, and psychological problems, such as anxiety and depression.
X0 (Turner) syndrome.
Turner syndrome (described in 1938 by Henry Turner) is a condition that affects only females, resulting when one of the X chromosomes is entirely (monosomy 45,X0) or partially missing. Turner syndrome occurs in 1 in 2500 born females and can cause a variety of medical and developmental problems, including short stature, failure of the ovaries to develop (estrogen deficiency, no menstruation, and infertility), poor breast development, and heart defects [39]. Most women with Turner syndrome are infertile due to oocyte loss that starts during embryonic life: they experience regular migration and mitosis of germ cells, but the oogonia do not undergo meiosis, and rapid loss of oocytes leaves the gonad without follicles early in life, and ovarian tissue appears as a fibrous streak. Most women with Turner syndrome require egg-donation treatment for infertility. Spontaneous pregnancies are seen in 2–5% of Turner syndrome patients and are most likely to occur in women with the mosaic form of this syndrome.
Y0 syndrome.
As the X chromosome carries about 800 protein-coding genes, some of which are critical for embryo viability, compared with the 70 genes on the Y chromosome, there is no known Y0 syndrome.
Autosomal translocation.
Autosomal translocations are 4–10 times more common in infertile males than in the fertile population [40–41]. Most translocations do not affect other tissues but can severely impair spermatogenesis. This effect may be due to the disruption of genes responsible for spermatogenesis or due to impaired synaptic complex pairing and segregation during meiosis [42–45]. Unbalanced reciprocal translocation carriers have a higher risk of producing unbalanced gametes or offspring with abnormalities. Both the chromosomes involved in translocation and the location of the breakpoints are supposed to be determining factors for the fidelity of synapsis, and thus the carrier’s fertility status [45]. Carriers of autosomal translocation always have a reduced number of gametes with an unbalanced karyotype [46].
Sex chromosome translocation.
Any part of the sex chromosome can translocate to autosomes like other chromosomal translocations. Sex chromosome translocations have direct consequences on genes required for germ cell differentiation. Translocations between the Y chromosome and autosomes are rare and can involve any part of the Y chromosome, every so often leading to abnormal spermatogenesis and hence infertility [47–48]. The suggested mechanisms for impaired fertility due to sex chromosome translocation are the transformed gene loci or impaired formation of the sex vesicle during meiosis. During the first meiosis of spermatogenesis, pairing between the X and Y chromosomes occurs in primary spermatocytes, resulting in a condensed sex vesicle. Sex chromosome pairing seems to be distinct from autosome pairing, as it is limited to the telomere pseudoautosomal regions (PARs), PAR1 (at Xp/Yp) and PAR2 (at Xq/Yq). Changes of pseudoautosomal sequences result in impaired pairing between the X and Y chromosomes, therefore indicating that there is a recombination event in the DNA homolog segment located in PARs during the first meiosis, which is essential to promote meiotic pairing and ensure sperm production [49].
Defects and mutations of the short stature homeobox gene (SHOX) in the PAR1 are related to the short stature of Turner syndrome and idiopathic growth retardation [50–51]. Translocations concerning a sex chromosome and an autosome are more likely to cause infertility than translocations involving autosomes.
Y Chromosome Microdeletions
Y chromosome microdeletions are found in 5–15% of infertile men with azoospermia and are a relatively common cause of male infertility [52]. Such deletions are characterized by azoospermia or severe (<1 × 106 sperm cells/ml of semen) to moderate oligozoospermia (1–5 × 106 sperm cells/ml of semen), with only rare cases of mild oligozoospermia (5–15 × 106 sperm cells/ml of semen). Males with Y chromosome infertility usually do not have obvious symptoms, although physical examination may reveal small testes and/or cryptorchidism, or varicocele. The incidence of Y chromosome microdeletions is estimated to be about 1:2000 to 1:3000 males [53–55]. Y microdeletion analysis is usually carried out by polymerase chain reaction (PCR) amplification of three loci, named the azoospermia factor (AZFa, AZFb, and AZFc loci), in the q arm of the Y chromosome (region Yq11). AZFc is the most commonly found (60%) deletion compared with AZFa (5%), AZFb (16%), or their combination (14%) [56]. This commonly found deletion is in part because AZFc is four times longer than AZFa [56]. Genes within the repeats, called the “amplicons” portion of the male-specific Y region, are expressed exclusively in the testis and are believed to contribute to the development and proliferation of germ cells. Multiplex PCR is a rapid method for the detection of submicroscopic Y chromosome deletions, which are undetectable by conventional cytogenetic analysis and conventional PCR.
The azoospermia factor comprises several genes such as USP9Y (ubiquitin-specific protease 9Y), DBY (DEAD box Y-linked), RBMY (RNA-binding motif Y-linked), PRY (PTPN-related gene on Y), UTY (ubiquitously transcribed tetratricopeptide repeat containing Y), CDY (chromodomain protein Y-linked), DAZ (deleted in azoospermia), and HSFY (heat shock transcription factor Y-linked). These genes are critical for spermatogenesis at different stages of germ cell development. Deletions of AZFa and AZFb are usually rare and are associated with Sertoli cell-only syndrome and maturation arrest, respectively [57]. Therefore, it has been proposed that patients with a microdeletion involving AZFa and AZFb should not undergo TESE. AZFa is expressed in spermatogonial stem cells and AZFb in premeiotic germ cells (primary spermatocyte). In contrast to AZFa and AZFb, partial or complete AZFc loss is related to a highly variable heterogeneous phenotype, ranging from oligozoospermia to azoospermia. Chromosome Y infertility is inherited in a Y-related manner; however, AZF region deletions in the long arm of the Y chromosome are usually de novo.
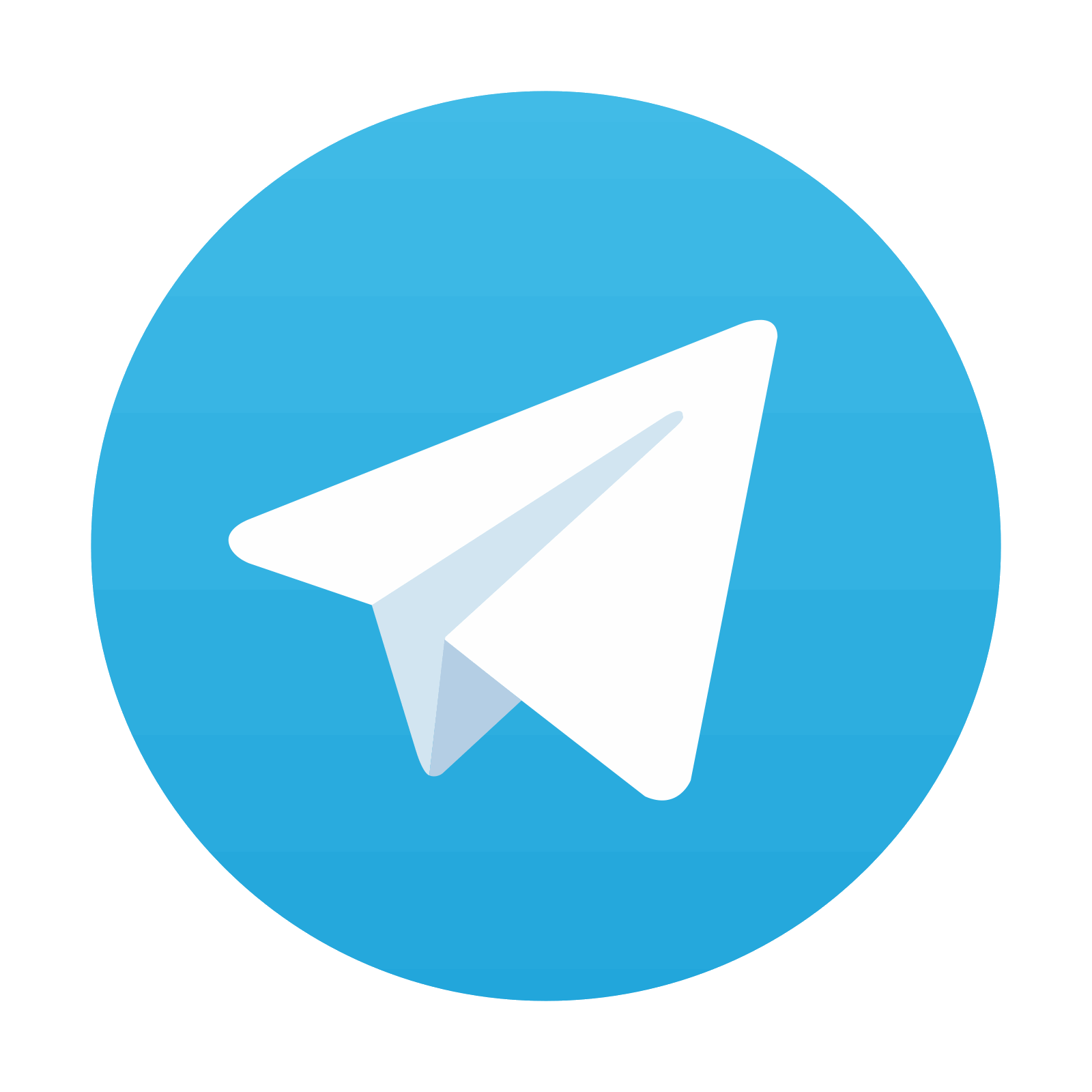
Stay updated, free articles. Join our Telegram channel
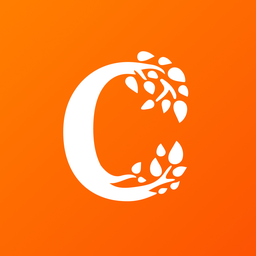
Full access? Get Clinical Tree
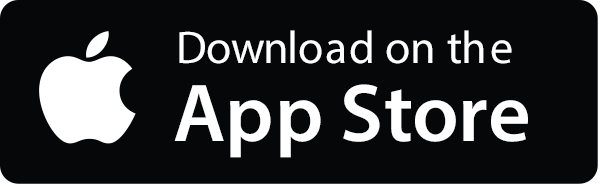
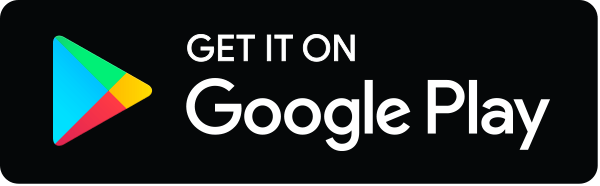