Abstract
In vitro maturation (IVM) systems have been developed to support the growth and development of oocytes, from their most immature stages to developmentally competent oocytes. The IVM technique has been in veterinary practice for a long time. The first reported study of IVM in humans dates back to 1965; however, the first pregnancy resulting from IVM in humans was only reported in 1991 and involved the use of donated oocytes from unstimulated ovaries from women undergoing gynecological surgery. A 1994 report described a pregnancy in an anovulatory woman with polycystic ovary syndrome (PCOS) after IVM of her oocytes.
In vitro maturation (IVM) systems have been developed to support the growth and development of oocytes, from their most immature stages to developmentally competent oocytes [1–2]. The IVM technique has been in veterinary practice for a long time. The first reported study of IVM in humans dates back to 1965 [3]; however, the first pregnancy resulting from IVM in humans was only reported in 1991 and involved the use of donated oocytes from unstimulated ovaries from women undergoing gynecological surgery [4]. A 1994 report described a pregnancy in an anovulatory woman with polycystic ovary syndrome (PCOS) after IVM of her oocytes [5]. Initial reports concentrated on the development of specific culture conditions [6], variations in stimulation and priming protocols [7], and patient selection [8], as well as fertilization techniques [9]. Traditionally, cycles of IVM are followed by intracytoplasmic sperm injection (ICSI) for fertilization, although similar fertilization rates have been reported for IVM-in vitro fertilization (IVF), rendering IVM-IVF an optional method, due to its cost-effectiveness and lower invasiveness [10]. Earlier studies that compared the results after IVM with those after conventional IVF (in vivo-matured oocytes) reported significantly lower pregnancy rates with IVM. The majority of the treatment protocols involved human chorionic gonadotropin (hCG) priming, which led to the early resumption of meiosis. The adoption of a “freeze-all” IVF protocol, with the transfer of a single blastocyst in a subsequent frozen embryo transfer cycle, has led to live birth rates that approximate those of traditional IVF cycles, with the avoidance of ovarian hyperstimulation syndrome (OHSS) [11]. These improvements have generated a renewed interest in IVM research, particularly for PCOS patients.
To develop IVM methodologies, we must fully understand the physiological processes that occur in vivo.
Oocyte Maturation In Vivo
The prolonged arrest in meiosis occurs in the primary oocyte (germinal vesicle [GV]-arrested oocyte at prophase I) before birth. Years later, meiosis is reinitiated just before ovulation and is again halted at metaphase II (MII) until fertilization. Fertilization activates the completion of meiosis and is followed by successive mitotic divisions of the newly formed embryo.
Before resumption of meiosis, the oocyte undergoes a growth phase, during which it accumulates both mRNA and regulatory proteins required for the completion of meiosis. Meiotic progression depends upon the precise control of these essential regulatory proteins and any alteration in this control could result in maturation failure. These proteins are controlled via regulation of their production, phosphorylation, localization, and degradation.
The extended meiotic arrest of the oocyte at the GV stage is maintained by several external factors, including cAMP, most of which are transferred to the oocyte from the cumulus cells (CCs) via gap junctions. Elevated cAMP levels downregulate meiosis promoting factor (MPF), which is a heterodimer, composed of p34cdc2 kinase (known also as CDK1) and cyclin B. Granulosa cells (GCs) produce natriuretic peptide precursor type C (NPPC) that binds the NPPC receptor (NPR2) on CCs, resulting in the production of cGMP, which is then transferred to the oocyte via gap junctions [12] to inhibit phosphodiesterase 3 A (PDE3A), thereby preventing hydrolysis of cAMP [13–14]. cGMP is also nitric oxide (NO) dependent, and supplementation of NO inhibits meiotic resumption [15].
The oocyte meiotic resumption is an incredibly complex chain of reactions (Figure 13.1). There are many molecular cascades maintaining meiotic resumption in the oocyte, all of which are orchestrated by multiple molecules produced by the pituitary gland and follicular cells. Meiosis reinitiation is stimulated by gonadotropins, luteinizing hormone (LH) and follicle-stimulating hormone (FSH), preovulatory peaks. While LH receptors are located on GCs and CCs [16], CCs have been shown to be insensitive to direct LH stimulation [17]. A surge in LH induces reprogramming of the mural GCs in the follicle and the expression of epidermal growth factor-like peptides, including amphiregulin and epiregulin, that are needed to propagate the LH stimulus from the mural GC to the CC [17]. FSH binds to its receptors on both GCs and CCs. The transition from GV to GV breakdown (also known as the metaphase I [MI] stage) is the first morphological marker of meiotic resumption in the oocyte. The breakdown of the nuclear envelope occurs about 2 hours after LH exposure [18]. LH interrupts cell-to-cell interactions in the ovarian follicle (expansion and disconnection of the cells). Connexin 43, the ovarian gap junction protein, is dramatically phosphorylated during the LH and FSH surges, leading to gap junction closure between CCs, thereby reducing the supply of cGMP to the oocyte within 2 hours. This event, in turn, leads to the activation of intra-oocyte PDE3A that reduces intra-oocyte cAMP. Disconnection of the gap junctions between cells also reduces the cAMP supply to the oocyte. A low level of cAMP reinitiates meiosis and oocyte maturation. LH also inhibits adenylate cyclase, leading to a drop in the production of cAMP, which has an added effect on oocyte maturation. cAMP acts as a primary negative regulator of meiosis by inhibiting p34cdc2 kinase dephosphorylation. Dephosphorylation of p34cdc2 kinase on Thr-14 and Tyr-15 by cdc25 results in MPF activation, which drives meiosis resumption via an increase in the activity of mitogen-activated protein (MAP) kinase (MAPK; MAPK1/2 known also as MEK1/2 and MAPK3 known as ERK) that remains elevated until the completion of the second meiotic division. Active MAPK is required to maintain the oocyte in the second metaphase arrest. The upstream regulator of MAPK in the oocyte is Moloney sarcoma (MOS) kinase, the product of the c-mos protooncogene (Figure 13.2). In most cases, the subsequent inactivation of MPF is brought about by the ubiquitin–proteasome pathway activated by anaphase-promoting complex/cyclosome (APC/C directs the destruction of cell cycle regulatory proteins at the metaphase–anaphase transition of first and second meiosis). APC/C activation results in a release of separase (also known as separin), which is necessary for chromatid separation (Figure 13.3). At the transition from stage MI to stage MII, actin-related protein 2/3 regulates migration of the MI spindle to the oocyte membrane, which is necessary for extrusion of the first polar body (PB).
Figure 13.1 Regulation of MPF inactivation and activation. Cx43; connexin 43; GVBD, germinal vesicle breakdown; MAP kinase, mitogen activated protein kinase; MOS kinase, Moloney sarcoma kinase; MYT1, myelin transcription factor 1; PKA, protein kinase A; PKB, protein kinase B.
Figure 13.2 Oocyte maturation and the arrest of meiosis at second metaphase.
A global analysis of the number of active genes detected in oocytes showed a progressive decrease in the number of genes expressed during oocyte nuclear maturation, with the lowest number of genes expressed in MII oocytes compared with GV or MI oocytes [16]. The translation of all three zona pellucida glycoproteins decreases dramatically during oocyte maturation, while genes involved in meiosis, such as MPF, APC/C, and spindle checkpoint complexes, are markedly overexpressed.
Full achievement of meiosis is only accomplished after fecundation because metaphase exit is prevented by the activity of cytostatic factor (CSF), which is only relieved by gamete fusion [16]. The endpoint of activation with any activating agent (i.e., penetration of the sperm cell into the oocyte) eliciting Ca2+ release is the physical destruction of cyclin B1, which leads to inactivation of p34cdc2 kinase and to a drop in MPF and CSF activity (CSF reduces cyclin B1 degradation) [19].
Approximately 8.6–15.2% of all infertility patients produce at least one meiotically incompetent oocyte. Women who are showing more than 25% immature oocytes suffer from significantly lower fertilization with lower clinical pregnancy rates [20]. Oocyte maturation failure is occasionally absolute, i.e., no mature oocytes are produced. Oocyte maturation arrest can occur at any of its development stages: GV arrest, MI arrest, or MII arrest.
Type I (GV arrest).
The activation of MPF controls the resumption of meiosis from the diplotene arrest. Morphologically, reinitiation of meiosis I and entry into the M phase is marked by the breakdown of the GV. Disruption of the key signaling events leading up to MPF activation results in the arrest of the oocyte before MI. In an animal model, cdc25b (-/-) female mice (cdc25 dephosphorylates and activates MPF) bear oocytes arrested in the GV stage [21]. Women who produce oocytes arrested at GV possess a defect in the signaling cascade responsible for the activation of MPF. Regulation of oocyte cAMP expression has also been found to be essential for oocyte maturation. PDE3A is primarily responsible for oocyte cAMP hydrolysis. PDE3A-deficient female mice are entirely infertile because ovulated oocytes contain increased cAMP levels and are arrested at the GV stage [22].
Type II (MI arrest).
Progression through meiosis MI to MII arrest requires chromosome condensation, the formation of the metaphase spindle, and chromosome alignment along with the first metaphase plate, followed by the separation and segregation of the homologous chromosomes. The completion of the first meiotic division is marked by the formation of the first PB. Oocyte arrest before the formation of the first PB is morphologically classified as MI arrest. MI arrest could occur because of either a defect in the signal transduction pathway mediating meiotic progression or any abnormality in the meiotic spindle, which results in activation of the spindle assembly checkpoint. APC/C mediates the metaphase-to-anaphase transition. Disruption of the critical signaling events leading up to APC/C activation results in the arrest of the oocyte at the MI stage. Targeted degradation of securin by APC/C releases separase, which cleaves cohesin and results in chromosome segregation [23]. APC/C also triggers the destruction of cyclin B, leading to the inactivation of MPF and exit from meiosis I [24]. Additional oocyte factors can be involved in MI arrest. The knockdown of H1foo (an oocyte-specific subtype of the linker histone H1) resulted in oocyte arrest at MI [25]. In vivo, H1foo function is mediated through methylation; therefore, it is possible that epigenetic modification of H1foo may result in altered gene expression and oocyte maturation competence. Mei1 and Mlh1 mediate recombination during meiosis [26] and Mei1-deficient oocytes demonstrate severe synapsis defects and unpaired and disorganized chromosomes and undergo MI arrest [27].
Type III (MII arrest).
MII oocytes are generally described as “mature” and arrested in MII; they are presumed to be capable of fertilization. However, there are subsets of women who produce oocytes with MII morphology that are not capable of embryo formation after fertilization. Some of these women may be carrying oocytes arrested at MII due to their inability to complete meiosis. They may be carrying immature oocytes arrested at any stage between first PB formation and MII, which match the MII morphological classification but are still unable to be fertilized. Arrest at MII has initiated inhibition of APC/C by Emi2 and maintained inhibition of the MOS/MAPK pathway and spindle assembly checkpoint by Emi2 [28]. Oocytes deficient in Emi2 protein are not able to form a spindle complex during the second meiosis and are arrested at MII [29]. Similarly, MOS-null mutant oocytes that progressed to MII could not complete second meiosis after fertilization [30]. Release from MII arrest is dependent upon sperm-specific phospholipase C zeta and downstream activation of APC/C [31]. APC/C activation results in the inactivation of MPF and the release of separase, which is necessary for sister chromatid separation [32]. Proteins involved in this pathway include calmodulin-dependent protein kinase II (CamKII) and protein kinase C (PKC). CamKII and PKC inhibitors block exit from meiosis, resulting in MII arrest [33]. Maturation arrest is characterized by incapacity of the oocyte to progress through meiosis due to either signaling errors or aberrations in chromosomal/spindle formation. These aberrations can be a reason for aneuploidy. Aneuploidy was found in 41.9% of oocytes after first meiosis (homologous chromosomes) and 37.3% of oocytes after second meiosis (sister chromosomes), with 29.1% of these oocytes having both first meiosis and second meiosis errors [34].
Although nuclear maturation and cytoplasmic maturation are linked, cytoplasmic maturation can occur independently of full nuclear maturation. During the initial growth phase of the oocyte, nuclear chromatin is decondensed and is transcriptionally active. As folliculogenesis progresses, the oocyte acquires meiotic competence, as identified by the condensing and nuclear association of chromatin, and the formation of microtubule organizing centers, necessary for spindle formation. Developmental competence requires a series of nuclear and cytoplasmic cellular events that take place alongside meiotic stages, to enable fertilization, DNA replication, and zygote ploidy.
At present, the use of donor oocytes is the only option available for women with oocyte maturation failure after several unsuccessful stimulation cycles.
Oocyte Metabolism during Maturation
Anaerobic glycolysis is a major pathway for glucose metabolism in the mammalian follicle, consuming glucose and producing lactate as the growing follicle undergoes luteinization [35–36]. The oocyte, however, has a low glucose metabolism capacity due, in part, to low phosphofructokinase activity [37]. Instead, CCs with high glycolytic activity metabolize glucose into pyruvate, which is transferred to the oocyte as an energy source. In the oocyte, pyruvate is converted, in the mitochondria, to acetyl CoA, which enters the tricarboxylic acid cycle and electron transport system to produce ATP [36]. The oocyte, in turn, ensures receipt of pyruvate by upregulating glycolytic genes in CCs [38].
During ovulation, the maturing oocyte supports the increased energy expenditure through mitochondrial oxidation of free fatty acids, a more efficient source of ATP than glycolysis [39]. Fatty acids within the cumulus–oocyte complex enter the mitochondria, where they are converted to acetyl CoA and continue to produce ATP through beta-oxidation [40]. With increased oxygen and pyruvate consumption during oocyte maturation, the oocyte also regulates the delivery of amino acids and cholesterol from CCs for other cellular processes.
Free radicals that can damage cellular macromolecules during follicle growth and oocyte maturation include reactive oxygen species (ROS) and reactive nitrogen species. High ROS levels and low antioxidant capacity in follicular fluid predict lower pregnancy outcomes following IVF [41–42].
Oocyte Maturation In Vitro
IVM offers a number of potential benefits when compared with standard IVF, principally related to the avoidance of high doses of hormonal stimulation with gonadotropins and gonadotropin-releasing hormone (GnRH) analogs. In patients with PCOS, this avoids the risk of OHSS [43]. Moreover, IVM can also be offered in other cases, such as gonadotropin-resistant ovary to stimulation by FSH [44].
For the follicle aspiration technique employed in an IVM cycle, most centers use a small-gauge size (16 or 17 gauge) with either a single- or double-lumen needle, with suction pressures in the range of 52–200 mm Hg [45–46]. The aspiration of oocytes, when the leading follicle is 10–12 mm in diameter, and the transfer of a single blastocyst-stage embryo performed under hormone therapy to assist endometrial development demonstrated excellent implantation and pregnancy rates [47]. To synchronize the development of the fertilized oocyte and endometrium, estradiol (E2) is first administered at a dose of 2 mg, three times a day, from the day of oocyte collection, followed by progesterone (P4) administration from the next day after oocyte collection. The dosage of P4 depends on the formulations, which include oral, vaginal, rectal, subcutaneous, and intramuscular preparations. The dosage of E2 depends on the endometrial thickness. If the endometrial thickness is less than 6 mm, 10–12 mg of E2 are given; if the endometrial thickness is between 6 and 8 mm, 8–10 mg of E2 are administered in divided doses.
The effectiveness of IVM relies on the successful synchronization of the meiotic and cytoplasmic maturation of oocytes [48]. There are several types of IVM: classic IVM (maturation of COC in vitro with gonadotropins from GV stage), hCG-primed IVM (truncated IVF, followed by IVM), and rescue IVM (maturation of denuded immature oocytes after regular IVF).
Sample of classic protocol for IVM: in vivo FSH priming (150 IU daily for 3 days) from day 3 of the cycle, then coasting for 2–5 days, followed by hCG priming. Since smaller follicles do not express LH receptors, they are insensitive to hCG. GCs respond to LH once follicles reached 9–10 mm, therefore CC-oocyte complexes retrieved from 10 mm follicles (dominance is not established at this stage) resulted in recovery with most oocytes at the GV stage. Recovered CC-oocyte complexes are cultured in medium with gonadotropins for 30–48 hours. IVM culture media are based on culture media, hormonal additives, and a source of protein. For successful resumption of meiosis, the combination of FSH with either hCG or LH in the culture media is necessary to promote the proliferation and expansion of the coronal cells and aid in the final stages of oocyte maturation. The combination of FSH with hCG improved clinical pregnancy rates and implantation rates in a randomized trial [49].
The timing of oocyte aspiration is not so critical when hCG priming is used; however, it is critical when no trigger is used. Improved clinical outcomes were obtained upon transfer of single vitrified warmed embryos in a non-hCG-primed IVM cycle to PCOS patients, compared with fresh embryo transfer [50]. These effects are mainly attributed to low endometrial receptivity in fresh embryo transfer cycles. Embryos transferred in a vitrified–warmed cycle are associated with clinical pregnancy rates identical to those after IVF cycles in women with PCOS [51]. The first IVM baby was born in October 2003. A 33-year-old woman became pregnant from an oocyte that had been matured in vitro, fertilized, frozen as a blastocyst, and then thawed [52].
Immature human oocytes are able to mature in vitro, particularly those from gonadotropin-stimulated ovaries; however, they are developmentally incompetent when compared with oocytes matured in vivo. Oocyte size is associated with maturation in most species examined, which may indicate that a specific size is necessary to initiate the molecular cascade of normal nuclear and cytoplasmic maturation. The loss of developmental competence in oocytes cultured to MII in vitro is associated with the absence of specific proteins. The presence of follicle support cells in culture is necessary for the gonadotropin-mediated response required to mature oocytes in vitro. Gonadotropin concentration and the sequence of FSH and FSH–LH exposure may be significant for human oocytes, particularly those not exposed to the gonadotropin surge in vivo. Exposure of immature oocytes to high FSH levels during IVM accelerates nuclear maturation and disrupts chromosomal alignment on the spindle during first meiosis, causing an increase in aneuploid MII oocytes [53]. The co-culture of CCs with non-mature oocytes improves the maturation of the oocytes, possibly due to the accumulation of a protein critical for spindle assembly [54]. Complete maturation of oocytes is essential for the developmental competence of embryos.
IVM is certainly no panacea for poor-prognosis patients with low levels of anti-Müllerian hormone (AMH) and antral follicle count (AFC). On the contrary, IVM success rates are highly correlated with AMH and AFC levels. IVM oocytes differ from in vivo-matured oocytes, including reduced protein content in the former compared with the latter oocytes [55]. In addition, differential gene expression was found between in vivo maturation and IVM [56]. Over 2000 genes are expressed at more than two-fold higher levels in IVM oocytes compared with in vivo-matured oocytes [57]. Transmission electron microscopy showed that IVM oocytes have normal ooplasm, with uniform distribution of organelles. However, large mitochondria–vesicle complexes partially replaced mitochondria–smooth endoplasmic reticulum aggregates in IVM oocytes. Vacuoles were found in all oocytes and were frequently associated with lysosomes. [58]. Multinucleation rates following ICSI also are higher in IVM than in in vivo-matured MII oocytes [59]. The IVM oocytes also had lower normal fertilization rates than the MII-aspirated oocytes [60]. In addition, embryos derived from oocytes that failed to mature in vivo under standard treatment of ovarian stimulation may show a different morphokinetic profile than their sibling oocytes aspirated at the MII stage after completing maturation in vivo [60]. Rescue IVM produced ~1.5 more additional embryos for transfer [61] compared with embryos from in vivo-matured oocytes. However, pregnancy rates with IVM remain lower than with conventional IVF, and higher miscarriage rates have been reported with IVM. As research of IVM expands, success rates are slowly improving, but still remain lower than regular IVF.
High rates of chromosomal aberrations have been reported in rescue IVM embryos [62]. A comparison of IVM with classic IVF and/or ICSI has found no differences in the incidence of congenital anomalies [63]. Although there is currently limited evidence of the long-term outcomes of children born following IVM, studies have demonstrated that the outcomes are similar to conventional IVF controls [64].
IVM suggests a valuable alternative for assisted reproductive technology in select patient populations, such as those at high risk of OHSS, delivering improved pregnancy outcomes and more efficient bench-time management compared with traditional IVM laboratory techniques. IVM is also significantly cheaper than conventional hormonal alternatives [43].
Follicle Maturation In Vitro
Primordial follicles can be grown to produce oocytes that can be fertilized. In a mouse model live offspring have been produced from such in vitro-grown oocytes [65]. A two-step culture system has been developed for human primordial follicle activation and growth to the second stage within cortical pieces [66]. Other groups have demonstrated the developmental potential of growing isolated human multilaminar follicles in vitro [67] and have confirmed that they can develop from the preantral to the antral stage in a two-step culture system, where they resume meiosis and reach MII [68]. An in vitro model of ovarian follicle development is characterized by accelerated follicular maturation, which is associated with improved developmental competence of the oocyte, compared with follicles recovered in vivo [69]. A four-step combined (in vitro follicle growth and oocyte IVM) culture system was developed. The system requires the removal of growing follicles, which are then cultured in a serum-free medium for 8 days (Step 1). At the end of this period, the secondary/multilaminar follicles are dissected from the strips, and intact 100–150 μm in diameter follicles are selected for further culture. Isolated follicles are cultured individually in serum-free medium in the presence of 100 ng/ml human recombinant activin A (Step 2). Individual follicles are monitored and after 8 days, and cumulus–oocyte complexes are retrieved by application of gentle pressure on the cultured follicles. Complexes with complete CCs and adherent mural GCs are selected and cultured in the presence of FSH and activin A for an additional 4 days (Step 3). At the end of Step 3, complexes containing oocytes more than 100 μm in diameter are selected for IVM in medium (Step 4). In vitro-grown human oocytes progress to MII after a total of 20 days in culture, starting from primordial/unilaminar follicles [70].
Significantly more genes involved in “response to stimulus,” “secretion,” and “extracellular matrix” are identified in CCs, suggesting that CCs are more active in cell-to-cell communication processes than the oocyte. Conversely, genes annotated “reproduction,” “ubiquitin ligase complex,” “microtubule-associated complex,” “microtubule motor activity,” “nucleic acid binding,” and “ligase activity” are significantly more frequently associated with genes overexpressed in oocytes, in agreement with the major processes involved in meiosis and implying attachment of microtubules to chromosomes and APC/C regulation [14].
APC/C regulates the migration of the MI spindle to the oocyte membrane, which is necessary for the extrusion of the first PB, which expresses the maturity status of the oocyte. An oocyte displaying a first PB is not necessarily expressed at the MII stage, because still immature telophase I oocytes may show a first PB as well. Both the proximity of the spindle to the oocyte cortex and the interchromosomal spacing within MII spindles influence PB size [71]. Wider spacing of chromosomes and loss of spindle contact with the oocyte cortex leads to extrusion of PBs of unusually large sizes compared with controls [72].
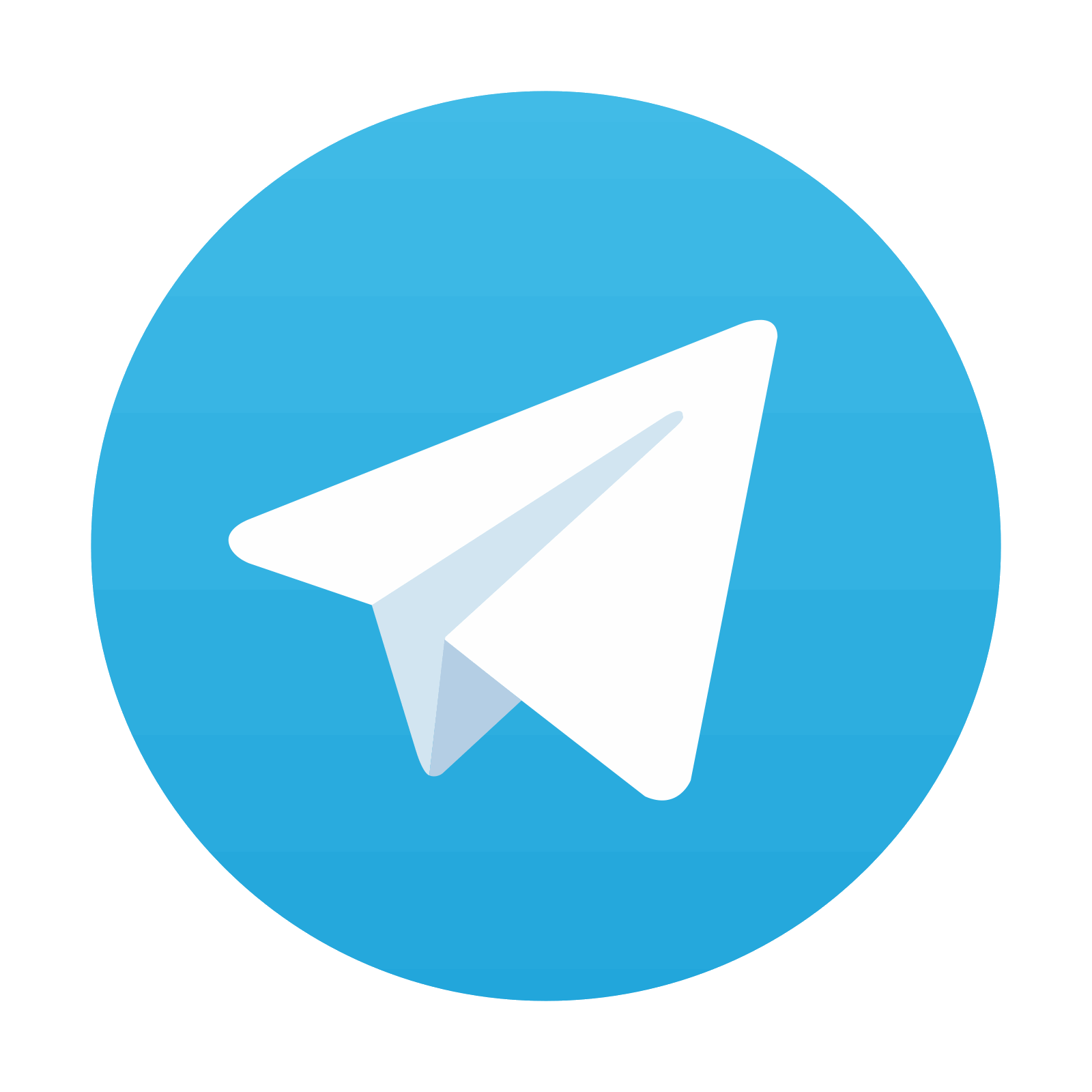
Stay updated, free articles. Join our Telegram channel
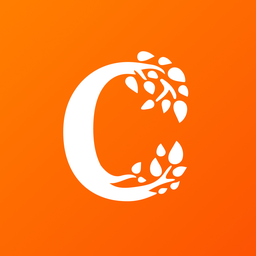
Full access? Get Clinical Tree
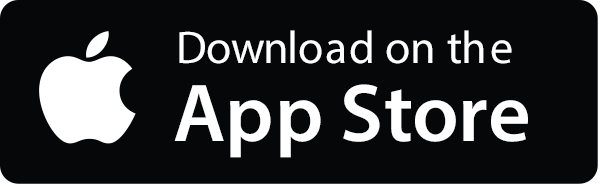
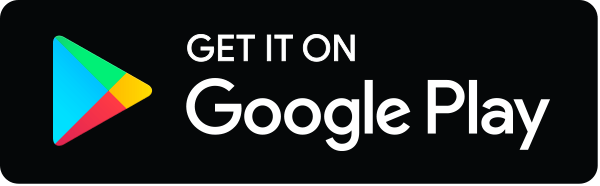