Abstract
Cryopreservation involves the cooling of cells and tissues to subzero temperatures to stop all biological activity and preserve them for future use. Initial cryopreservation methods were ineffective as simple cooling techniques led to cellular damage due to the altered concentration of solutes within the cells, ice formation, and excessive dehydration.
Cryopreservation involves the cooling of cells and tissues to subzero temperatures to stop all biological activity and preserve them for future use. Initial cryopreservation methods were ineffective as simple cooling techniques led to cellular damage due to the altered concentration of solutes within the cells, ice formation, and excessive dehydration.
Cryopreservation is an adjacent and useful procedure of assisted reproductive technology (ART). In 1948, Dr. Chris Polge serendipitously discovered that glycerol, in a mislabeled bottle of sugar solutions, is an effective cryoprotective agent for sperm. The first human delivery from frozen sperm was reported in 1953. In the 1970s, additional cryoprotectants such as propanediol (propylene glycerol [PrOH]), dimethyl sulfoxide (DMSO) and ethylene glycol (EG) were identified and established to minimize cellular damage (Figure 12.1). Since the 1970s, oocytes and embryos of more than 30 mammalian species have been successfully cryopreserved, bringing about the birth of millions of normal offspring. The first human birth from a cryopreserved embryo was in 1984 [1]. Oocytes and embryos have been cryopreserved by slow freezing or vitrification methods. The slow-freezing method uses relatively low concentrations of 10% cryoprotective additives (CPAs), cooling rates higher than 1°C/min, and warming rates higher than 250°C/min. In contrast, vitrification, or the reversible transition of a liquid into an amorphous noncrystalline glass [2], uses high CPA concentrations (30–40%), saccharides as supplements, cooling rates much higher than 2000°C/min, and very speedy warming rates. Often, extremely high cooling rates more than 10 000°C/min are used.
Figure 12.1 Types of regular cryoprotective additives.
Mechanism of Cryopreservation
One of the major causes of cell destruction during freezing and thawing is the formation of intracellular ice crystals. Treatment of the cells with cryoprotectants forces osmotic water movement and leads to dehydration of the cell. While shifting water out of a cell may help reduce the amount of intracellular ice crystal formation, it may result in osmotic shock and a detrimental change in volume. The basic concepts of slow cryopreservation are to protect the cells from the effect of intracellular ice crystal formation, drastic changes in solute concentrations, and cellular dehydration at both high and low temperatures. Cryoprotectant is thought to protect cells by reducing or eliminating lethal intracellular ice formation, by stabilizing intracellular proteins, and by moderating the impact of concentrated intracellular and extracellular electrolytes [3]. One major factor affecting the response of a cell to freezing is the ratio of its surface area to volume. In general, the larger the cell, the slower the cooling process must be to ensure survival. Additionally, at temperature around 0°C, special care must be taken to avoid extreme fluctuations in cell volume during CPA equilibration, as rapid volume change can immediately damage cells and make them more sensitive to stress during subsequent cooling or thawing procedures [4]. At the same time, exposure to CPAs should be minimized due to their potentially toxic effect. For example, DMSO induces plasma membrane pore formation and initiates caspase-3-independent apoptotic processes upon translocation of apoptosis-inducing factor from the mitochondria to the nucleus [5]. Cryoprotectant toxicity can be lowered by reducing the temperature of exposure, but this may require a longer exposure time. Although many investigators attribute cellular damage during freezing solely to the toxicity of the CPAs, others argue that cellular damage is due entirely to osmotic shock.
When aqueous solutions are frozen, water is removed, causing the components within the cell to become increasingly concentrated as the temperature falls; the reverse occurs during thawing. As the cells are frozen, they respond osmotically to massive changes in extracellular fluid concentrations. It has also been claimed that cells can be damaged upon extended exposure to high electrolyte concentrations, excessive cell dehydration, and the mechanical effects of external ice.
Vitrification is a cryopreservation method in which the biological material and the surrounding liquid solution become an amorphous glass-like solid, free of all crystalline structures when plunged in liquid nitrogen (LN2) [2]. Vitrification requires much higher concentrations of cryoprotectants, which may elicit toxic and osmotic effects. Since the development of the first vitrification solution in the 1970s, which contained DMSO, PrOH and acetamide [2], various CPAs have been found to be effective. The most extensively used CPAs are composed of permeable agents that penetrate the cell (such as EG, DMSO, PrOH or glycerol) as well as non-permeable, low-molecular-weight, non-penetrating CPAs (such as raffinose, sucrose, trehalose, egg yolk citrate, albumin). Also, CPA solutions contain high-molecular-weight polymers such as Ficoll (PM 70 or 400).
Many “open” and “closed” carrier devices are currently utilized to load the biological material before storage in LN2, and their design can indirectly impact outcomes by causing alterations in the cooling and warming rates. However, the variety of vitrification methods, carrier devices, and hypertonic CPAs may introduce complexities when oocytes or embryos at different stages of development are transferred to other in vitro fertilization (IVF) centers that routinely use different techniques to perform vitrification.
The success of a vitrification protocol is based on effective dehydration upon cell exposure to hypertonic conditions, as well as on the penetration rate of a CPA, which should be sufficient to generate an intracellular environment that will vitrify and remain vitrified for a defined cooling–warming rate. Also, CPA removal causes successive phases of shrinkage and re-expansion, due to movement of water and CPA across the cell membranes.
In the vitrification process, oocytes or embryos are exposed to a non-vitrifying CPA solution, containing only permeable CPAs, and then to a vitrifying CPA solution, containing both permeable and non-permeable CPAs. The CPA concentration and composition vary between commercial kits. During exposure to the non-vitrifying CPAs, the cell immediately adjusts its osmolarity by losing water and shrinking. The CPAs then enter the cell slowly due to their low membrane permeability [6]. The duration of exposure to the non-vitrifying solutions at a defined temperature is of critical importance, as it determines the intracellular CPA concentration. Depending on the CPA used and the type and developmental stage of the biological material, exposure time may range between 3 and 15 minutes. The biological material is then exposed to vitrifying CPAs for 30–90 seconds. This solution causes further cellular dehydration, which concentrates the intracellular CPA. The overall goal of this procedure is to create an intracellular environment that will remain vitrified for a defined cooling–warming rate.
The transition of water and CPAs across cell membranes is crucial for cell survival during cryopreservation, and the degree of membrane permeability depends upon the stage of embryo development (Figure 12.2). While it is possible visually to detect the shrinkage and swelling of cells under a stereomicroscope, it is not possible to estimate the proportion of CPA that enters the cells. Only full expansion of oocytes or embryos, representing full equilibration with CPA, may be assessed. Water and CPAs can move across the plasma membrane of oocytes and embryos by simple, slow diffusion through the lipid bilayer membrane, in which case, permeability is low and temperature-dependent energy activation is high. In parallel, aquaporins, a variety of water channels expressed by mammalian oocytes and embryos, play a role in CPA transport [7]. Permeability via the channels is high and temperature-dependent energy activation is low [8]. The movement of water across each unit of the cell surface as a function of time is a function of the cell volume. Oocytes and zygotes have the lowest surface/volume ratio compared with an embryo, so they are less effective than cleavage-stage embryos at losing water and taking up CPAs, and thus are more susceptible to intracellular ice formation if the exposure time to CPAs is not well adapted [9]. The ideal duration of exposure to CPA solutions may differ between stages of development. For example, in ultra-rapid vitrification, an average time of 5–15 minutes is necessary to protect oocytes from cryoinjury, while a maximum 5-minute exposure is required for collapsed blastocysts. Also, each CPA has its own cell penetration characteristics. During slow freezing, each CPA is intended to be adapted to different stages of embryonic development: PrOH for zygote and cleavage-stage embryos, DMSO for cleavage-stage embryos, and glycerol for day 5 embryos. Similarly, during vitrification, mixtures of DMSO/EG or PrOH/EG are used for oocytes as well as for the early stages of cleavage embryo development and blastocysts. In morulae and blastocysts, glycerol, EG, and DMSO move rapidly through the cell membrane by facilitated diffusion through channels, whereas PrOH enters only slowly by simple diffusion [8].
Figure 12.2 Mechanism of preparation (dehydration) of embryo for cryopreservation.
The transformation of a solution into a glassy state occures when the temperature is very rapidly lowered to below the glass transition temperature. To reach ultra-rapid cooling rates of more than 20 000°C/min, open embryo carriers (e.g., Cryoloop, Cryoleaf, Cryotop, Cryolock) were designed to allow direct contact of the biological material with LN2 (Figure 12.3). The advantage of ultra-rapid cooling is that although the cells are exposed to increasing CPA concentrations for a short time, enabling the extraction of the intracellular water, only a limited amount of CPA permeates the cells. Another alternative is hermetically closed carrier devices, allowing full isolation and protection of the sample from direct contact with LN2 during the cooling procedure and subsequent long-term storage. In a closed system, the specimen is not allowed to come in contact with the LN2. Therefore, a carrier is obligatory to deliver the maximum heat transfer rate to the contained specimen.
Figure 12.3 Process of vitrification (A) Transfer of embryo on the tip of carrier device. (B) Plunging of carrier device into LN2 followed by closing with device cover.
Cryopreservation of Embryos (Zygote/Cleavage-Stage/Blastocyst)
Since the first birth from a cryopreserved embryo, cryopreservation has been considered a routine and important component of IVF treatment.
Zygote cryopreservation.
It has been suggested that freezing at the zygote stage provides for improved results compared with freezing at the cleavage stage [10]. This is probably due to the reason that a zygote is a single cell, like the oocyte, but without the complexities arising from the sensitive meiotic spindle. Zygote vitrification is a routine method in ART [11], with survival rates at least as high as with slow freezing. However, vitrification and warming can have a significant impact on zygote morphology; about 15% of zygotes do not show pronuclear appearance after warming, which has been associated with poorer outcomes [12]. This type of morphological change is uncommon after a slow freezing.
Cleavage embryo cryopreservation.
Cryopreservation of embryos optimizes the IVF cycle by enabling the storage of all excess embryos, and reducing the risk of multiple pregnancies. Frozen embryos also allow the patient to achieve pregnancies without requiring subsequent stimulation of a new IVF cycle. In cycles with hyperstimulated ovaries, cryopreservation can reduce the risk of ovarian hyperstimulation syndrome [13]. Cryopreservation of the embryo has been successfully achieved at the cleavage (day 2 or day 3) and blastocyst (day 5) stages. Embryos are initially maintained in an equilibration solution comprising Ham’s F-10 medium, supplemented with 7.5% EG, 7.5% DMSO, and 20% patient serum, for 5–15 minutes, at room temperature (~20–25°C) [14]. After the initial shrinkage and recovery, the embryos are aspirated and placed in the vitrification solution (15% EG, 15% DMSO, and 0.5 M sucrose) at room temperature (~20–25°C), for at least 50 seconds but no longer than 60 seconds. After visually confirming that cellular shrinkage has occurred, the embryos are aspirated and placed on the tip of the loading device (carrier) and directly in contact with the LN2.
Embryo survival rates are the primary parameter used to assess the efficiency of a freezing program. The survival rate is accepted as a function of the number of vital embryos thawed, as assessed by morphological evaluation immediately after thawing. The final selection of thawed embryo for transfer can be based on a range of factors, including the number of intact blastomeres and resumption of mitosis. Pregnancies from damaged embryos have been achieved and required a threshold of 50% blastomere survival [15]. Later reports demonstrated the importance of obtaining fully intact embryos, which were associated with significantly higher implantation rates than embryos with cell loss [16].
Blastocyst cryopreservation.
Blastocyst vitrification is conventionally performed on collapsed or non-collapsed blastocysts. Applying assisted hatching before blastocyst vitrification allows better permeation of the cryoprotectants and better blastocoele dehydration [17]. No statistical differences in survival, implantation, or clinical pregnancy rates were noted between blastocysts that had undergone laser pulse opening or micro-needle puncture [18]. Collapsed blastocysts have to be exposed to equilibration solution, which generally contains 7.5% DMSO/EG, for 2–5 minutes, while non-collapsed blastocysts require a ~10-minute incubation in DMSO-free glycerol solution. After that, samples are incubated in vitrification solution (15% DMSO/EG and 0.5 M sucrose) for 1 minute. At the time of vitrification, the blastocyst is placed in a loading device surrounded by vitrification media. The device is then plunged into and stored in LN2. Embryos frozen at the expanded blastocyst stage exhibited equal viability, implantation potential, and pregnancy outcome [19].
During the thaw, the embryo is subjected to a three-step warming procedure. First, it is immediately immersed in 1 M sucrose for 1–1.5 minutes, then transferred to 0.5 M sucrose two times for 2 minutes each, and then washed three times in buffer MOPS or HTF (human tubal fluid) for 3 minutes each and then cultured in medium.
Frozen–thawed blastocysts undergo multiple morphological changes that include the collapse of the blastocoele cavity along with cellular lysis and degeneration. Morphological assessments of thawed blastocysts are necessary to estimate the survival of individual cells and the whole embryo. By visual examination of the extent and locale of cellular degeneration, a skilled embryologist can estimate the proportion of viable cells. Blastocysts that develop to at least grade 2 in expansion, with at least grade B inner cell mass (ICM) and TE, and with no signs of degeneration, according to Gardner’s grading system [20], are vitrified on day 5. All remaining embryos are assessed on day 6 and vitrified if they reach at least grade 4 in expansion, at least grade B ICM and TE both, and show no signs of degeneration. These assessment criteria are associated with successful cryopreservation of at least one blastocyst in 36% of cycles. The pregnancy and implantation rates using vitrified blastocysts are comparable to those associated with the use of fresh blastocysts [21]. Biopsied embryos cryopreserved at the blastocyst stage have demonstrated better survival following vitrification than following slow freezing.
The vitrification outcome (embryo survival) is highly operator dependent and requires entirely different skills than those needed for slow freezing. For example, the embryologist must rapidly handle the embryos in micro-volumes of extremely viscous media. Also, because there are a variety of loading devices available, specific training on the use and storage of each device and standardization of quality control procedures is mandatory.
Singletons born after cryopreservation of embryos (cleavage-stage or blastocyst) had a significantly higher birth weight than both those conceived by fresh embryo transfer and naturally conceived singletons [22]. The reason for this occurrence could be explained by the epigenetic effect of increased methylation at the imprinting control region of the H19 gene in embryos during cryopreservation.
Cryopreservation of Oocytes
Oocyte cryostorage is indicated in various situations, for both subfertile and fertile patients. For example, it is recommended for women at risk of losing their reproductive function due to oncological treatment or premature ovarian failure. Women, who wish to delay motherhood for “social reasons,” including career, absence of a partner, or adverse social and economic conditions, could also benefit from oocyte vitrification. Christopher Chen reported the first successful attempt at freezing and thawing a human oocyte in 1983, and the first human birth from standard inseminated frozen oocyte was reported in 1986 [23].
Oocytes are extremely sensitive to cryoinjury, and the meiotic spindle, cytoskeleton, cortical granules, and zona pellucida (ZP) are at particular risk. The initial success of oocyte cryopreservation was limited by the fragility of the metaphase II (MII) oocyte, rooted in its size, water content, and chromosomal arrangement. Oocyte cryopreservation requires that cells tolerate three nonphysiological conditions: exposure to molar concentrations of CPAs, cooling to subzero temperature, and removal of almost all liquid cell water or its conversion to a solid state. Vitrification of human oocytes has proven effective, and usually superior compared with standard slow freezing, with functional survival of cryopreserved oocytes, i.e., the ability to go through fertilization and embryo development, approaching 100%. The ability of the extracellular solution to form glass when cooled at high rates is considered essential to successful oocyte cryopreservation. The key to survival is the vitrification capacity of the oocyte contents.
The first step is to equilibrate the cells with a CPA. One of the keys to the success of oocyte cryopreservation appears to be reaching adequate permeation of CPAs across the oocyte membrane. Because EG readily permeates oocytes, its use lessens the likelihood of osmotic shock when oocytes are diluted out of even very concentrated solutions.
High-quality MII oocytes suitable for cryopreservation are colorless and of regular shape, with regular ZP and a small perivitelline space without debris, homogeneous cytoplasm, and no vacuoles or granulations [24–25]. Also, the presence of an intact, round or ovoid polar body (PB) with a smooth surface should be considered a selection criterion [26]. Furthermore, an excessive extension of the culture period before freezing could affect oocyte competence after cryopreservation, since oolema permeability changes over time [27]; therefore, oocyte cryopreservation should be carried out as soon as possible after retrieval (~3–4 hours). Current best practice denudes oocytes immediately before cryopreservation. For slow freezing, this should be completed within 38 hours after human chorionic gonadotropin (hCG), with intracytoplasmic sperm injection (ICSI) performed 2–3 hours post-thaw to allow the spindle reorganization. For vitrification, oocytes should be vitrified 38–40 hours post-hCG, with ICSI performed at the equivalent of 40–41 hours post-hCG (i.e., between 1 and 3 hours post-warming).
MII oocytes are sensitive to cryoinjury because the meiotic spindle is acutely temperature sensitive. Transient cooling of oocytes to 20°C can irreversibly disrupt the spindle apparatus, whereas rapid depolarization occurs when the temperature is lowered to 0°C [28–29]. The appropriate organization of spindle microtubules is crucial for the correct alignment and segregation of chromosomes. Oocyte freezing can, therefore, increase the rate of aneuploidy during extrusion of the second PB, through nondisjunction of sister chromatids. In addition to the cytogenetic effect of cryopreservation, there is an increased risk of parthenogenetic activation of the oocytes after thermal shock and exposure to CPAs.
There are some oocyte behaviors during vitrification that may predict the outcome of a later warming procedure [14]. For example, during exposure to the equilibration solution, the oocytes should be completely shrunken within 3–6 minutes, depending on the dilution of the equilibration solution. From this time, oocyte shape starts to recover, with full recovery expected within 9–15 minutes. For human oocytes, full-size recovery seems to be highly important for the success of the warming procedure: if they are still smaller than before the process, an additional equilibration time is strongly recommended. During exposure to the vitrification solution, the oocytes initially shrink very quickly and float on the surface. They then partially recover but are not able to completely reshape before being loaded onto the carrier and vitrified.
Cryopreserved specimens should be transported between clinics/laboratories at a temperature below -132°C (ideally below -150°C), which should be verified by a temperature logging device.
Social oocyte preservation.
Delayed marriage and late childbearing have led to a dramatic decline in birth rate and population. Nothing has changed in women’s physiology that can support the deliberate delay of childbearing. Pregnancies and deliveries should ideally be completed before a woman reaches the age of 35, at which point, fertility tends to drop at a faster rate. Women who freeze their eggs before the age of 35 preserve the fertility at the age of freezing. Social oocyte freezing is a procedure lacking indication. The target population is all single women of almost all age groups. The intense debate about the role of “social” egg freezing repeats many of the assertions made initially about IVF itself. Women who wish to freeze their eggs for their future use face many hurdles. Vitrification of oocytes and the accumulated experience with frozen donor egg banks have shown that “young” frozen eggs thawed, fertilized, and transferred as blastocysts have the same reproductive potential as “fresh” eggs [30]. The recommended number of eggs to be frozen to give a fair chance of success differs with age. Statistics show that, in general, only 1 out of 20–25 eggs collected (4–5%) will result in the delivery of a baby [31]. The regular number of eggs collected in a single cycle is 8–12, requiring two to four cycles at the cost of 6–15 000 US$ per cycle, plus variable annual storage cost, to freeze 20–25 mature eggs [32]. To suggest that women who freeze their eggs are likely to be too “choosy” when seeking a suitable partner is demeaning of women who decide to avail themselves of this technology. Those who do choose to freeze at an ideal time, i.e., under the age of 35 (or even earlier), may be faced with the intolerable dilemma of allowing their eggs to stay frozen or creating embryos with donor sperm, which may then be stored for additional years.
Thawing Oocytes/Zygotes/Embryos
Warming is a “reversal” of the cooling process and aims to rehydrate the cell and to remove the cryoprotectant that has permeated the cell. In order to avoid osmotic shock, gradual, stepwise removal of cryoprotectant is essential [33]. When frozen cells are warmed rapidly, melting of the cell suspension is equivalent to a rapid dilution of the CPA that turns out to be concentrated during the freezing process. The rapid influx of water into cells as the extracellular milieu begins to melt can cause an osmotic shock at subzero temperature. Sensitivity to osmotic shock is, therefore, a function of the cell’s permeability to water and solutes. This shock can be decreased by the use of a nontoxic, impermeable substance as an osmotic buffer, such as sucrose [34]. Warming is usually initiated by the immediate transfer of the device with a sample to a prewarmed environment.
The first warming step for oocytes is generally performed at 37°C, in a 1 M sucrose solution for 1 minute (Figure 12.4); as soon as the oocytes are released from the carrier, they tend to float and appear “vitreous.” In the next warming solution, generally 0.5 M sucrose for 2 minutes, the oocytes shrink and look as if clear and very bright. During the following warming steps, 0.25 M sucrose and/or subsequent three sets of washing for 3 minutes each, the oocytes slowly recover their original shape. If the oocytes appear dark or flat or quickly recover their shape at the first stages of warming, they are possibly damaged and will degenerate.
When thawing cleavage-stage embryos, some remain intact, while others are fully degenerated or partially survive, with a mix of necrotic and normal blastomeres within the same embryo. The embryo transfer of partially damaged cleaving embryos is associated with decreased implantation rates as well as lowered pregnancy rates when compared with the transfer of fully intact embryos [35]. Integration of assisted hatching with lysed cell removal has been proposed to improve the implantation potential of such embryos [36]. If fragments can be reabsorbed by embryonic cells of fresh embryos, the same thing or the same mechanism can be observed in thawed intact embryos [37]. In contrast, degenerated cells in frozen–thawed embryos will not or cannot be reabsorbed by the remaining living cells of the embryo; therefore, the removal of necrotic cells could be recommended [38]. Poor-quality thawed embryos (<50% intact blastomeres after thawing) resulted in a zero implantation rate [39].
Survival of human oocytes/zygotes/embryos after cryopreservation can be affected by their stage of development, their quality, or biophysical factors resulting from the cryopreservation procedure used. The combined elements of freezing and thawing represent a dynamic process during which many physical and chemical factors, such as osmotic and hydrostatic pressure, intracellular ionic content, pH, and temperature, fluctuate over a wide nonphysiological range and may impact genomic integrity.
After warming, viable blastocysts re-expand and are usually incubated for 4–6 hours to regain their vitality before being transferred. Re-expansion is the sign of viability. The timing of blastocyst re-expansion is an important predictor of vitrified–warmed blastocyst transfer outcomes; the earlier the blastocyst expands, the better it is expected to perform transfer [40].
Cryopreservation of Ovarian Tissue
In prepubertal girls, adolescents, and women who cannot delay the start of chemotherapy or radiotherapy, cryopreservation of ovarian tissue is the only available fertility preservation option. The ovarian tissue is obtained before the onset of the gonadotoxic anticancer therapy and can be autotransplanted to the patient after she has been cured of her disease. This technology may also be used to preserve fertility in girls with mosaic Turner syndrome, who experience premature menopause, and subsequently lose their ability to conceive during the regular fertile years [41]. This procedure could be useful for female-to-male transgender [42].
Different types of cells exhibit different optimum warming rates. This phenomenon is especially relevant to the cryopreservation of ovarian cortex, a tissue composed of diverse types of cells, each with its characteristic shape, size, and permeability properties. Therefore, cooling and warming conditions that are optimum for one cell type within the ovarian cortex may be harmful to others. More importantly, the survival of frozen tissues is dependent on the rate and method by which CPAs are removed from cryopreserved tissues. The recently reported successful cryopreservation of whole human ovaries represents a new method in considering whole ovary autotransplantation for the clinical way of cryopreservation [43].
Ovarian tissue can be picked up at any time during the menstrual cycle. Cryopreservation of ovarian tissue means cryopreservation of ovarian cortex due to the follicle localization in the cortex of the ovary. The primordial and primary follicles are located at a mean depth of 271 μm in infants and 460–500 μm in women. The majority of secondary follicles are located less than 1000 μm from the ovarian surface (mean depth: 639 μm). In women with premature ovarian insufficiency (POI), the mean depth of primordial and primary follicles is 566 μm, whereas 70% of secondary follicles are located at depths of more than 1000 μm [44]. These measures suggest that an optimum ovarian tissue thickness of less than 1 mm should be chosen for cryopreservation in regular patients and thicker ovarian cortices, which include secondary follicles, should be subjected for cryopreservation in POI patients (Figure 12.5). Primordial follicles, which comprise about 90% of the follicular population of each ovary, are less cryosensitive than maturing follicles, mature oocytes, and embryos, primarily due to their structureless cytoplasm and cryosensitive cytoplasmic components, lack of ZP, fewer granulosa cells, as well as the low metabolic rate associated with their dormancy. Primordial oocytes appear to be less sensitive to cryoinjury than mature oocytes as they are smaller, lack a ZP and cortical granules, and are relatively metabolically quiescent and undifferentiated.
Figure 12.5 Ovarian cortex cryopreservation by portions of fragments.
Various protocols are used worldwide for the cryopreservation of ovarian tissue for fertility preservation purposes. The efficiency and efficacy of the majority of these protocols have not been extensively evaluated. A practical method for the preservation of the human ovarian cortex is based on Gosden’s slow-freezing protocol and involves equilibration of thin slices (~1.5 mm thick) for 1 hour at 4°C, in a freezing solution containing cryoprotectant plus sucrose [45]. This protocol ensures a maximal surface area for rapid CPA (EG or DMSO) penetration. Ovarian specimens are cooled at 0.5°C/min to approximately -55°C and then at 2°C/min to approximately -140°C, and plunged into LN2. Then, ovarian tissue is stored in LN2 at -196°C.
Vitrification of ovarian tissue fragments can be completed using a two-step vitrification procedure, with equilibration first in 7.5% DMSO, 7.5% EG, and 20% synthetic serum substitute (SSS), followed by incubation in a final vitrification medium containing 15% DMSO, 15% EG, and 20% SSS [46]. Another successful vitrification protocol involves equilibration of slices in a solution of 7.5% DMSO and 20% SSS followed by a vitrification solution containing 15% DMSO, 15% EG, 20% SSS, and 0.5 M sucrose [47].
Ovarian cortex specimens are thawed rapidly by being swirled in a water bath at ~25–37°C, and the CPA is diluted out of the tissue by repeated rinses with fresh medium, under continuous agitation. DMSO is a known epimutagen and EG has been reported to be toxic at high concentrations, therefore, it has to be removed in a short time. DMSO requires a more prolonged and more elaborate thawing procedure than EG, as well as continuous dilution. CPA traces can remain in the warmed/thawed ovarian tissue before transplantation [48] and their impact on the mother and the baby must be considered.
After cryopreservation/thawing, the majority of ovarian follicles are in the primordial or primary stages [49]. Most of the follicles (more than 90%) in the antral stage show some signs of degeneration after freezing and thawing [49]. Stromal cells, which are essential for the neovascularization of an ovarian graft after autotransplantation [50], are more vulnerable to the damaging effects of the freeze–thaw procedure than follicles.
A comparison of slow-freezing and vitrification of ovarian tissue showed that the number of secondary follicles was lower in the vitrified group. At the same time, AXIN1 (a gene encoding a cytoplasmic protein which contains a regulation of G protein signaling domain and an axin domain) expression was significantly lower in slow-frozen samples compared with vitrified samples [51]. The expression of apoptotic genes, excluding CASP3, was significantly reduced in slow-frozen samples. Conversely, BAX/BCL2 expression was significantly higher in vitrification versus slow freezing. Follicles in slow-frozen samples displayed nuclear and cytoplasmic β-catenin staining, while control and vitrification groups only showed β-catenin protein in the cytoplasm. Slow freezing results in superior preservation, regardless of the type of follicle. Although slow freezing of ovarian cortical fragments is currently used in clinical settings, vitrification offers a more cost-effective and ice-free alternative for preserving ovarian tissue. Improvements in the outcome of transplantation of ovarian tissue are further anticipated, particularly concerning revascularization.
Transplantation of ovarian tissue restores both fertility and endocrine function. Transplantation of ovarian tissue resulted in a remarkably consistent return of menstrual cycling and normal follicle-stimulating hormone (FSH) levels within 4–5 months [52]. The finding that as FSH decreased, ovulation resumed, with anti-Müllerian hormone (AMH) initially rising to high levels followed by a return to very low levels, indicated that a massive over-recruitment of primordial follicles led to subsequent depletion in the ovarian reserve [52]. Despite low AMH levels, the grafts sustained ovarian function for long periods of time. Transplanted slices of ovarian cortex continued to function normally for several years, likely due to the smaller ovarian reserve and the decreased rate of primordial follicle recruitment. However, cryopreserved stock of ovarian cortex slices may play an important role as a reservoir for fertility preservation for a long time. The first human live birth from orthotropic transplantation of frozen ovarian tissue was reported in 2004, with another successful live birth achieved in 2005 [52–53].
The disadvantage of the ovarian cryopreservation method includes the risk of malignant cell contamination. The solid fibrous tissue of the ovarian cortex controls follicle development and represents a relatively inhospitable location for cancer cells. Storage of ovarian tissue for cancer patients has a risk of reintroducing malignant cells in the tissue graft [54]. Most cancer diseases do not display specific cancer markers. Therefore, in most cases, it is not possible to test for potential malignant cell contamination in the ovarian tissue. Furthermore, even if markers were available, testing of one or two ovarian pieces for cell contamination does not necessarily represent the real status of cancer cells in the remaining ovarian pieces due to be transplanted.
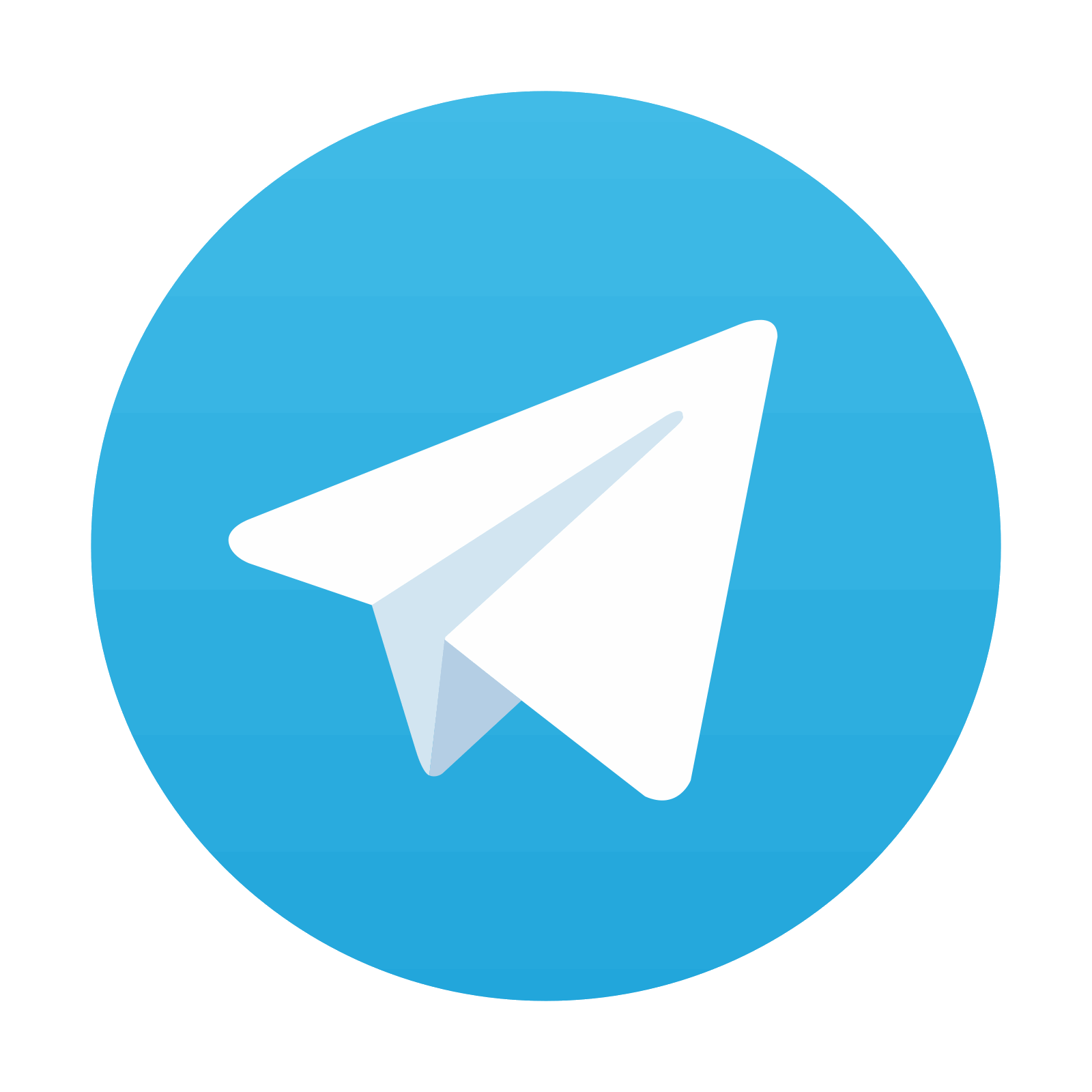
Stay updated, free articles. Join our Telegram channel
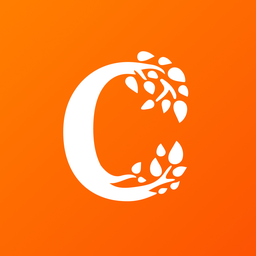
Full access? Get Clinical Tree
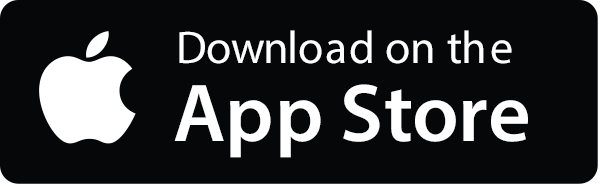
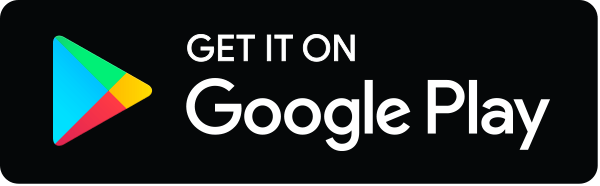