Abstract
Global tissue culture media were first developed by Ludwig and Ringer, nearly 150 years ago. These were simple salt solutions, simulating the properties of serum. The second generation of culture media, developed in the 1970s, mimics the female reproductive tract environment. A new, third generation of media was more improved and provided optimal growth conditions in vitro. The shift from home-brewed culture media to commercially manufactured media has contributed to improvements in laboratory and clinical outcomes, eliminating inconsistencies, manufacturing errors, and batch-to-batch variability.
Media Preparation for the Embryo Culture
Global tissue culture media were first developed by Ludwig and Ringer, nearly 150 years ago. These were simple salt solutions, simulating the properties of serum. The second generation of culture media, developed in the 1970s, mimics the female reproductive tract environment. A new, third generation of media was more improved and provided optimal growth conditions in vitro. The shift from home-brewed culture media to commercially manufactured media has contributed to improvements in laboratory and clinical outcomes, eliminating inconsistencies, manufacturing errors, and batch-to-batch variability. In 1963, Ralph Brinster designed the method of culture for oocytes and embryos in small droplets of culture medium covered by a layer of paraffin oil [1]. Later on, this “micro-drop” method had become the most widely used for the culture of mammalian embryos in vitro. By the mid-1990s, most IVF laboratories adopted the “closed” under-oil culture system, which has provided a more stable and more suitable culture environment for embryonic growth. Variations of the second-generation media called sequential and third-generation simplex optimized derived media are still in use at present and seem effective in supporting the development of human embryos through 6 days of culture in vitro. Culture for human embryos improved over the past decade by adding of essential amino acids.
After the compaction stage (day 4 of embryo culture), embryos have different requirements of amino acids and energy molecules compared with the cleavage stages (days 1–3). For example, the main energy source provided in an early-stage medium is lactate and pyruvate, as opposed to glucose for embryo culture from late day 3 with the onset of embryo compaction [2]. In addition, essential amino acids and metabolites, such as citrate and malate, are almost completely omitted from media used for early embryo culture, but are included in media used for embryos that have undergone compaction. Also, the chelating agent EDTA is included in early embryo culture media, but omitted from media used for extended culture, based on the assumption that this compound is toxic to blastocyst-stage embryos [3–4]. Solubilized ions, similar to those detected in the oviduct and uterine fluids, are the most abundant chemical constituents of culture media, to support human embryo cleavage and blastocyst formation in vitro. Together with purified water, ionic constituents contribute mainly to the osmolality of a medium. Media at a range of 250–290 mOsm can acceptably support mammalian embryo development [5]. Osmolality higher than 300 mOsm can induce deleterious changes in cell volume. Based on the knowledge of specific media requirements for the optimal embryonic development conditions, companies developed universal one-step media suitable for all embryo stages (Figure 10.1). Media from different manufacturers vary little in their composition of conventional ionic, protein supplements, and the main energy substrates: pyruvate, glucose, and lactate, which regulate mammalian embryo metabolism [6].
Figure 10.1 Comparison between one-step and two-step culture medium.
Amino acids are found in human reproductive tract fluids [7] and are essential regulators of embryo development. Glutamine was found to support the development of human embryos to the morula and blastocyst stages and increase energy metabolism. Taurine and glycine have been shown to act as osmolytes and, therefore, may help minimize the stress induced by osmotic fluctuations. Protein sources in culture medium play a role in preventing embryo adhesion to the culture plate and also may act as organic osmolytes and pH buffers. However, protein and amino acid metabolites in culture medium may negatively affect embryo development. Supplementation of culture media with protein showed ammonium accumulation (ranging from 8.4 mM to 138.6 mM), primarily from the amino acid glutamine metabolism by the embryo with the highest level at day 4 of incubation, the level that might inhibit embryo development and have a possible risk for fetal defects [8]. For this reason, embryo culture media have reduced amount of the amino acids and contain the more stable dipeptide forms of glutamine.
The addition of growth factors such as leukemia inhibitory factor, epidermal growth factor, insulin-like growth factor-I (IGF-I), and the cytokine granulocyte-macrophage colony-stimulating factor (GM-CSF) has been shown to support the development of human embryos to the blastocyst stage [9–12]. Furthermore, IGF-I and GM-CSF stimulated the development of the inner cell mass (ICM).
The majority of human embryo culture systems use a bicarbonate/carbon dioxide-buffered medium to maintain a physiological pH of 7.2–7.4. The combination of 25 mM sodium bicarbonate with 5% CO2 gas atmosphere results in a pH of 7.45 at sea level. An increase of carbon dioxide concentration up to 6% leads to decrease of pH till 7.37. These conditions are optimal for embryo growth and are highly recommended. Extracellular pH (pHe) in the culture medium is recognized to be a critical factor in establishing intracellular pH (pHi). pHi regulates protein conformation, glycolysis, and many of the other critical metabolic and transport processes in the embryo. The embryo can regulate its pHi, specifically acidosis, via the HCO3−/Cl− exchanger; however, even slightly fluctuations of pHi during 3 hours lead to the disorganization of mitochondria and actin cytoskeletal elements [13]. Therefore, appropriate and stable pHe is a crucial factor of the embryo culture system.
The temperature of media varies throughout the lab, and can be affected by the dish manufacture or media volume used. For example, a decrease in the temperature of media culture from 37°C to 36.5°C was associated with a significantly higher cleavage rate, but a lower blastocyst formation rate on day 5. For this reason it is recommended to use media warmed to 37°C [14].
Oxygen concentration is also a crucial factor in embryo culture. Atmospheric oxygen concentration of 20% is highly detrimental to the embryos of all mammalian species studied, negatively affecting metabolism, and the proteome and gene expression. In contrast, the oxygen concentrations in the reproductive tract of mammalian species have been reported to range between 1.5% and 8%. The culture of mammalian embryos at low concentrations of oxygen (5–7%) is thought to minimize the formation of embryotoxic reactive oxygen species. Reduction of oxygen concentrations to 5% did not affect blastocyst development, but increased total cell number compared with the culture at 20% O2 [15].
Media for fertilized oocyte and/or embryo culturing should be prepared and carbon dioxide/oxygen-saturated in an incubator for approximately 6 hours before use. A dish with prepared culture medium is covered with mineral oil to prevent medium evaporation under carbon dioxide/oxygen-saturated conditions at 37°C. The carbon dioxide/oxygen molecules pass through the oil–medium interface until the oil becomes saturated with carbon dioxide/oxygen. The thermodynamic transition of gas molecules through oil and its saturation takes approximately 4 hours, or more, depending on the type and volume of oil in the dish/plate. Usually, after 6 hours of incubation, the plate with medium covered by oil is ready for use. However, mineral oil may have a negative effect on the cultured embryo. It was shown that lipid-soluble materials are absorbed by mineral oil from the medium, and toxic compounds are released into the medium from the oil, which may lead to adverse effects [16]. For example, mineral oil peroxidation negatively affects media and embryo development. Conditions such as incubation time and high temperature can increase the peroxidation of mineral oil.
The embryo is sensitive to both chemical and physical signals within its microenvironment, which can play a significant role in its development and post-transfer events. These chemical and physical factors include oxygen, ammonium, volatile organics, temperature, pH, volume of oil overlay, medium volume/embryo density, exposure to atmospheric (20%) oxygen, and the accumulation of toxins in the media due to the static and undynamic nature of culture.
Some IVF laboratories culture embryos separately, one embryo per drop of medium, while others culture in groups of three to five embryos per drop. Increasing the fertilized oocyte density to two or four oocytes/culture drop does not affect the fertilization rate and embryo formation [17]. Culture of embryos in groups may have an advantage due to the secretion of growth-promoting factors, which act in an autocrine–paracrine fashion. The benefits of group embryo culture are in debate [18–19]. Embryo-to-embryo communication may not always be positive. The poor-quality embryos possibly exert a negative influence on the development of good-quality embryos in the same culture group [20].
The co-culture of embryos with somatic cells (granulosa cells, cumulus cells) has been reported to improve embryo and blastocyst development, decrease fragmentation, and improve pregnancy and implantation rates [21–22]. Somatic cells may enhance embryo development by providing trophic factors [23]. In summary, the gain in knowledge of both the physiology of the embryo and the environment of the oviduct and uterus leads to improvements in culture media and culture conditions.
Day 1 of Culture (Zygote)
Preparation for division begins when two haploid pronuclei (pN) disappear and syngamy occurs within 20–34 hours after fertilization (Figure 10.2). Mitotic division occurs approximately 35–36 hours after fertilization and results in the formation of two diploid blastomeres.
Figure 10.2 Zygote.
During the first hours of development after fertilization, the differentiated germ cells, i.e., the oocyte and the sperm cell, must be reprogrammed into a totipotent state. This process ensures that the newly formed zygotic genome can subsequently drive the differentiation of all the diverse cell types of the adult. The reprogramming relies on maternally supplied RNAs and proteins stockpiled in the oocyte. The zygotic genome remains transcriptionally silent while reprogramming takes place. As the paternal centrioles pull the chromatids apart, a channel appears between the two poles, and the zygote formed one-cell embryo cleaves into a two-cell embryo at approximately 35–36 hours postfertilization. Early entrance into the first cell division has been used as an indicator of embryo viability. Embryos that had undergone early cleavage within 25 hours of fertilization were associated with higher pregnancy rates [24]. However, for the embryo to continue developing after this initial reprogramming phase, the zygotic genome must be expressed. After the sperm has fertilized the oocyte, the centriole and microtubules arising from the sperm cell bring the female and male pN into juxtaposition [25]. Normally fertilized oocytes are spherical and have two polar bodies (PBs) and two pN. Male and female pNs appear virtually simultaneously at ~6.2 hours postfertilization. However, while the female pN always forms cortically and near the site of emission of the second PB, the initial position of the male pN is cortical, intermediate, or central (15.2%, 31.2%, and 53.6%, respectively) [26]. Pronuclear alignment occurs 16–18 hours postfertilization and failure of this process is indicative of lack of one or more fertilization events. The pN and nucleoli show various presentations [27], named “Z-score” (Z1–Z4, where Z1 is a higher score and Z4 is a lower score), which reflect the chromosomal status and developmental inferiority of the embryo (Figure 10.3). “Z-scoring” is determined by size, number, and distribution of nucleoli. Zygotes with three to seven even-sized nucleoli per nucleus have a high chance to grow to embryos with greater developmental potential. There have been several attempts to determine embryo viability based on the location and alignment of the nucleolar precursor bodies in the nuclei [27–28]. Appearance of “touching” p. 16–18 hours after fertilization is associated with a higher viability score. The sperm-derived centriole and microtubules arising from fertilization are responsible for the alignment of pN. Pronuclear alignment relative to the PB axis, which positions the nucleoli, has also been assessed as an indicator of embryo viability. Also, it has been suggested that the size variation between the two pN may indicate chromosomal anomalies and limited developmental potential [29]. Before syngamy, pN can rotate within the ooplasm, directing their axes towards the second PB [30]. Embryos failing to achieve an optimal pronuclear orientation may exhibit cleavage anomalies that can manifest as poor morphology, uneven cleavage, or fragmentation. Chromatin from the sperm and the egg is unified to create an entirely new genome. It remains unclear whether activation of the zygotic genome is instructive to the changes in the underlying chromatin or the changes in chromatin are required for genome activation. However, these processes are intimately linked. Two distinct genomes (paternal and maternal) undergo different processes as they are brought together in the zygote. In many species, the paternal genome is repackaged through the exchange of histones for protamines. In the transcriptional silence that follows fertilization, the genome is repackaged back to histones and reprogrammed to prepare the embryo to give rise to a new individuum. The transition from silence to widespread gene expression requires precise regulation. This regulation is accomplished through several coordinated processes, in which transcription factors play a central role. After fertilization, translational upregulation promotes the accumulation of genome-activating transcription factors, which direct chromatin remodeling in reprogramming contexts, helping to erase the previous cell identity (oocyte) while creating a new one (embryo).
Figure 10.3 Classification of zygotes by pronuclei and nucleoli according to Z-score.
It is unknown when and how molecular and morphological symmetry breaking emerges and how these two elements integrate spatially and temporally to control the genetic and epigenetic networks that direct lineage fate and pattern formation in the embryo. The molecular and physical circumstances under which distinct stem cell types can self-organize to form tissues, organs, or even artificial embryos in vitro are also unknown and it is vital that these be defined.
Tripronuclear or tetrapronuclear (or multi-pronuclear) zygotes form triploid (or multiploid) embryos and some regain a diploid karyotype by the expulsion of a set of chromosomes in a nucleated fragment [31]. Nondisjunction in meiosis I or meiosis II of oogenesis results in an extra set of maternal chromosomes, a state termed digyny. Nondisjunction in meiosis I or meiosis II of spermatogenesis results in an extra set of paternal chromosomes, a state known as diandry. Digyny is a triploid zygote in which the extra haploid set is of maternal origin (like retention of the second PB or binucleate oocyte), while diandry is a triploid zygote in which the extra haploid set is of paternal origin. Giant oocytes might be a possible source of digynic triploidy. It is not recommended to transfer or cryopreserve the embryos developed from giant oocytes or tripronuclear zygotes [31–32]. A monopronuclear zygote may be diploid; some of them may develop to a blastocyst and term after transfer [33–34]. It is suggested that monopronuclear zygotes be rechecked for multiple pN within a few hours and cultured to the blastocyst stage for possible transfer. However, the use of one pN zygotes, and zygotes showing no pN or more than two pN are not recommended by the European Society of Human Reproduction and Embryology (ESHRE).
Day 2 of Culture (Embryo Two to Four Cells)
Embryo score can be evaluated at high magnification (at least 200×, preferably 400×) using an inverted microscope with Hoffman or equivalent optics. Embryos are optimally at the four-cell stage of development by 45–46 hours postfertilization (day 2) [35]. Slowly developing embryos may still be at the two-cell stage at this time point. Some individual cells of the embryo may be asynchronous in their cell division, resulting in embryos with uneven cell numbers. It is accepted that transition from maternal to embryonic gene expression occurs between the four-cell and the eight-cell stage, i.e., during the first 48 hours after fertilization, the embryo primarily relies on maternal transcripts rather than its activated genome [36].
Number and size of blastomeres and embryonic fragmentation score are morphological features used to assess embryo quality [37–38] and which stand as the basis of the embryo classification system (grade 1 A to grade 4C), in which the numbers and letters represent fragmentation (<10%; 10–30%; 30–50%; >50%) and blastomeres (size and shape), respectively. In addition to these criteria, blastomeres can exhibit normal nucleation (one per blastomere), no nucleus, or multinucleation. Multinucleated embryos are associated with lower clinical pregnancy and live birth rates than single-nucleated embryos [39]; they are not associated with increased occurrence of congenital anomalies and chromosomal defects. It was suggested that blastomere multinucleation is a consequence rather than a cause of aneuploidy [40]. Fragmentation is the extrusion of the plasma membrane, including the cytoplasm of an embryo, into the extracellular region and appears to be a natural occurrence in human embryos [41]. Mitochondria are the most abundant structures found in the fragments [42]. The mechanisms causing fragmentation are not fully understood, although it has been speculated that developmentally lethal defects or apoptotic events may cause the process. Fragmentation of less than 10% is associated with a 23% live birth rate, 10–25% fragmentation with an 11% live birth rate, and more than 25% fragmentation with an only 1% live birth rate [43]. Fragment removal from the embryos does not improve embryo quality [42].
Day 3 of Culture (Embryo Six to Eight Cells)
By 54–56 hours postfertilization (day 3), embryos are optimally at the eight-cell stage of development (Figure 10.4) [35]. Embryos dividing either too slowly or too quickly may have metabolic and/or chromosomal defects (Figure 10.5). It was reported that more than 60% of the embryos that arrest between the pronuclear and the eight-cell stage are chromosomally abnormal [44]. Similarly, embryos exhibiting irregularly shaped blastomeres and severe fragmentation are considered poor-quality embryos and show a higher incidence of chromosomal abnormalities [45] on day 3 as well as on day 2 (Figure 10.6). Abnormal slow cleaving (two to six cells on day 3) and rapidly cleaving embryos show a higher incidence of chromosomal aneuploidy than embryos showing normal cleavage kinetics [46]. On day 3, embryos may contain blastomeres with more than one nucleus and are chromosomally abnormal compared with nonmultinucleated embryos [47].
Figure 10.4 Development stages of cultured embryo.
Figure 10.5 The uptake and excretion products of cultured embryo.
Figure 10.6 Classification of embryo by blastomere count, blastomere size, and fragmentation rate.
On day 3, embryo cells begin to become heterogeneous (first decision for differentiation to be trophectoderm cells or ICM later) [48–50]. Also, on day 3 postfertilization, there is an increase in cell–cell adhesion and partial loss of definition between single blastomeres of embryos that have initiated compaction. During compaction and with the establishment of cell–cell adhesions, blastomeres undergo a rearrangement process leading to communication between blastomeres signaling procession to the next stage – morula.
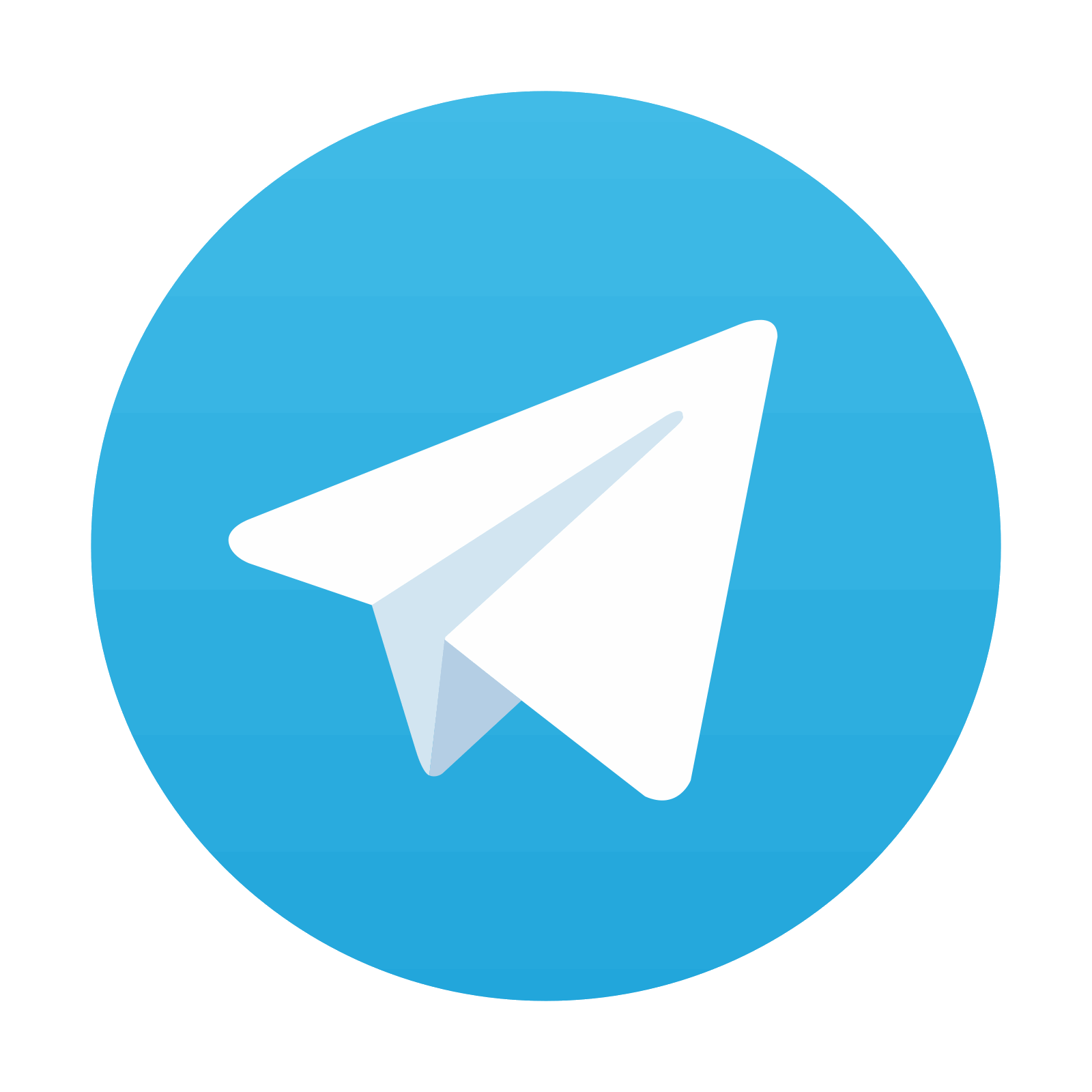
Stay updated, free articles. Join our Telegram channel
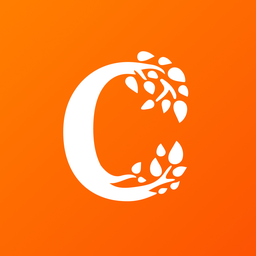
Full access? Get Clinical Tree
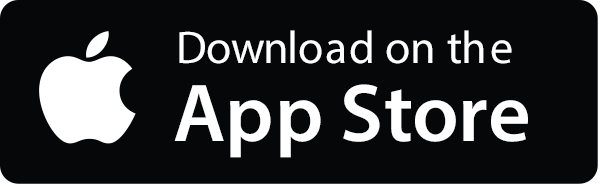
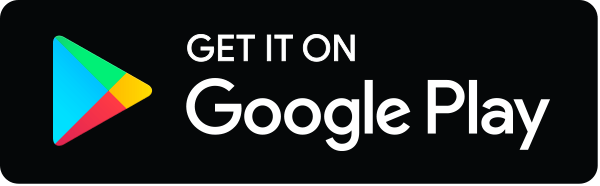