, Pierre-Henri Jarreau2, 3 and Brian P. Kavanagh1
(1)
Departments of Anesthesia and Critical Care Medicine, Hospital for Sick Children, University of Toronto, 555 University Avenue, Toronto, ON, M5G 1X8, Canada
(2)
Service de Médecine et Réanimation néonatales de Port-Royal, Hôpitaux Universitaires Paris Centre Site Cochin, 123, Bd de Port-Royal, 75014 Paris, France
(3)
Neonatal Intensive Care Unit, Université Paris Descartes, Assistance Publique-Hôpitaux de Paris, Fondation PremUP, Paris, France
33.1 Introduction
Mechanical ventilation saves the lives of those who would otherwise rapidly die from respiratory failure. Very early in its use it became clear, however, that mechanical ventilation could lead to adverse effects (i.e., ventilator-associated lung injury, VALI).
In 1939, Macklin reported that excessive alveolar distention led to the rupture of the junction between the alveolar wall and the vascular sheath, resulting in the tracking of air into the mediastinum, subcutaneous tissue, and/or pleural cavity (Macklin 1939). In the 1960s, it was shown that high tidal volumes (V T) and the absence of positive end-expiratory pressure (PEEP) adversely affect lung compliance and surfactant function (Faridy et al. 1966; Greenfield et al. 1964). During the polio epidemics in the 1950s, it was occasionally observed that patients ventilated with such injurious parameters developed lung lesions similar to those seen in the infant respiratory distress syndrome, i.e., dense cellular infiltrates, edema, and hyaline membranes; this presentation was termed “respirator lung.” Later, the acute respiratory distress syndrome (ARDS, at that time termed adult respiratory distress syndrome) was described with similar morphology (Ashbaugh et al. 1967), but the role of mechanical ventilation in contributing to the injury was not appreciated.
Most of the patients in the initial case reports were suffering from underlying lung disease, and it remained unclear to what degree the ventilator contributed to the pathological picture observed at necropsy. This difficulty persists today in the clinical setting. The term ventilator-associated lung injury (VALI) accounts for this difficulty and describes the assumed additional lung injury inflicted by a mechanical ventilator in patients with underlying lung disease (as opposed to the expression ventilator-induced lung injury [VILI], used to describe the lung injury induced exclusively by the ventilator, usually in animal models). However, the relative contributions of the underlying disease (e.g., ventilator-associated pneumonia) and the injury caused by the ventilator are never fully known.
ARDS has an annual incidence of roughly 75 per 100,000 (Rubenfeld et al. 2005) with an overall mortality in adults of 60 % (Ferguson et al. 2005), and in children, mortality may be in the range of 22 % (Flori et al. 2005). Because of the impossibility of dissecting out the contribution of the mechanical ventilator to the lung injury in the clinical setting, most evidence in the field comes from laboratory-based studies (i.e., VILI), using short-term animal models.
Does VILI or VALI present differently in children than in adults? There is some evidence that lungs of children and infants have a lower susceptibility to high ventilator settings compared to adults (Copland et al. 2004; Kornecki et al. 2005). Nevertheless, the overall risk of adverse effects from mechanical ventilation is high in younger (specially preterm) infants because of the small lung size (Jobe and Ikegami 1998), highly compliant chest wall, and immaturity of surfactant and defense mechanisms, in addition to associated comorbidities (see Sect. 33.3.3).
This chapter summarizes the current knowledge on the mechanisms of VILI, the clinical presentation, discusses predisposing factors, and outlines conventional and novel therapeutics useful in the prevention and management of lung injury during mechanical ventilation.
33.2 Lung Injury in the NICU
Pierre-Henri Jarreau4, 5
(4)
Service de Médecine et Réanimation néonatales de Port-Royal, Hôpitaux Universitaires Paris Centre Site Cochin, 123, Bd de Port-Royal, 75014 Paris, France
(5)
Neonatal Intensive Care Unit, Université Paris Descartes, Assistance Publique-Hôpitaux de Paris, Fondation PremUP, Paris, France
Educational Aims
-
Understanding the main physiological factors that are implicated in neonatal VLI
-
Taking these into account when modifying ventilator settings
-
Knowing the treatment concepts and ventilator strategies that might help to prevent neonatal VLI
The development and large-scale implementation of mechanical ventilation in the field of neonatology has dramatically improved the survival rate of many high-risk neonates, especially preterm infants. As with all treatment modalities, mechanical ventilation has been accompanied by certain complications. The consequences in the form of long-term sequelae essentially concern very preterm infants, and this chapter will therefore focus on bronchopulmonary dysplasia (BPD) or chronic lung disease of prematurity. This disease was unknown before the introduction of mechanical ventilation for premature newborns, and mechanical ventilation was initially considered to be the main cause of BPD. This was probably true in the first descriptions, but host factors, particularly severe immaturity, now appear to be major factors, which explains the relative stability of the incidence of BPD despite advances in respiratory care of these babies.
33.2.1 Predisposing Risk Factors
The lung of preterm infants is subjected to the same mechanisms of injury as the lung of older children and adults, but these mechanisms are exacerbated due to the particular state of development of the lung. Briefly, the formation of alveoli begins around 35 weeks of pregnancy, but extends into postnatal life. Extremely preterm infants have to breathe and often need to be ventilated while their lungs are still at the canalicular or early saccular stage of development. This abnormal situation leads to alveolar developmental arrest due to interferences with developmental mechanisms at multiple levels. Alveolarization involves the regulated expression of several genes and biological factors in various lung cell types (Bourbon et al. 2005) that act synergistically at a precise time of lung development. Many studies, based on animal models or human disease, indicate abnormal expression of gene products essential for the control of distal pulmonary development, induced by oxygen, mechanical ventilation, or airway inflammation as these injuries occur in a particular period of life.
The specific characteristics of respiratory mechanics of preterm infants, discussed in another chapter, may increase the risk of lung injury. In particular, surface tension is increased during the first hours of life (Greenspan et al. 2004) and is very high in the case of surfactant deficiency, responsible for respiratory distress syndrome (RDS), drastically reducing lung compliance and preventing the infant from maintaining functional residual capacity. In addition, infants with RDS have a lower total lung capacity (TLC), and thus, the lung volume between FRC and TLC may be low, increasing the risk of volutrauma (Attar and Donn 2002). This surfactant deficiency makes the preterm lung more susceptible to overdistention and atelectrauma leading to subsequent injury (Jobe 2006). Additionally, distribution of exogenous surfactant may be heterogeneous, which may be responsible for uneven ventilation and atelectasis. Surfactant deficiency also results in pulmonary edema (Jobe 2006).
The relative deficiency of preterm infants in antioxidant systems makes them very susceptible to reactive oxygen species (ROS)-induced injury, even at low partial pressure of oxygen. In fact, several antioxidant enzymes are upregulated late during fetal development to help newborns adapt to an environment with a relatively high level of oxygen. Thus, premature infants lack sufficient levels of antioxidant enzymes because this upregulation of antioxidant enzymes occurs during the last 3 months of pregnancy (Asikainen and White 2004; O’Donovan and Fernandes 2004).
Other factors like antenatal inflammation, persistent ductus arteriosus, lung fluid retention, and malnutrition may participate or worsen the consequences of neonatal lung injury.
33.2.2 Mechanisms of Lung Injury in the NICU
The main purpose of the lungs is to ensure gas exchange, which requires inward flow of fresh gases obtained by a pressure gradient between the mouth and the lung. During spontaneous ventilation, this gradient is ensured by a negative pressure mainly induced by contraction of the diaphragm. Expiration is passive by a recoil force of distended elastic elements of the respiratory system. During mechanical ventilation, the pressure gradient (except for negative pressure ventilation) is created by the ventilator, which imposes a pressure and a volume on the lung which are primarily responsible for lung injury by inducing barotrauma and volutrauma (Attar and Donn 2002). In addition to these mechanical factors, oxygen appears to be a major factor inducing arrested alveolarization. Airway inflammation associated with or induced by these factors also constitutes a major feature.
The few published autopsy studies have identified several common features in all infants with severe “old” or “new” BPD. Since the systematic use of surfactant and better control of all factors of lung injury, airway lesions and extensive fibrotic lesions are no longer observed or remain only minimal. On the other hand, signs of arrested alveolar development, clearly present on the first histological descriptions, now represent the almost exclusive lesion of BPD (Jobe 1999).
33.2.2.1 Mechanical Ventilation: Barotrauma, Volutrauma, and Atelectrauma
Experimental data show that both high peak pressures (barotrauma) and high tidal volumes (volutrauma) can cause lung injury. In the early 1990s, Dreyfuss et al. demonstrated that markers of lung injury are mainly linked to high tidal volume rather than to high pressures (Dreyfuss and Saumon 1998). To these two factors must be added atelectrauma, linked to alveolar instability, which is the result of repeated collapse and reopening of alveoli and respiratory bronchioles during the respiratory cycle (Schmolzer et al. 2008). These repeated reinflations from atelectasis impose a high shear stress on the alveoli. They also alter surfactant structure and function (Miller and Carlo 2008).
These mechanical factors may affect normal development by disturbing the expression of genes involved in normal development. Mechanical stretch of fetal lung epithelial cells activates various signaling pathways, resulting in transient or progressive increases in gene expression (Copland and Post 2007). Experimental data in preterm animals (lambs and baboons) or in species in which alveolarization is essentially postnatal (rodents) confirm these effects. Elastogenesis was disrupted, and elastic fibers displayed abnormal features in a lamb model of BPD (Bland et al. 2007b) or in ventilated mice (Bland et al. 2007a, 2008). Elastin is crucial for septal development (Bourbon et al. 2005), and elastic tissue is altered in BPD (Thibeault et al. 2000). Other extracellular matrix components such as matrix metalloproteinases are involved in lung development and are altered in BPD, at least in human neonates (Danan et al. 2002b). In ventilated preterm baboons, pulmonary microvasculature is abnormal with a decrease of certain angiogenic factors such as VEGF (Maniscalco et al. 2002). This disrupted vasculature has been confirmed in human preterm infants with BPD (Thibeault et al. 2004). Other growth factors involved in lung development can be involved in neonatal lung injury. Fibroblast growth factor-7 (FGF7 or keratinocyte growth factor) has been shown to be higher in the first 5 days of life in human preterm infants who survived without BPD (Danan et al. 2002a). This opens the way to adjuvant therapies which may be proposed in order to prevent VILI. The preventive effect of FGF7 on VILI has been demonstrated in an adult rodent model (Welsh et al. 2000), but at the moment, this protective effect has not been demonstrated in neonatal experimental models in which alveolarization is not restored (Franco-Montoya et al. 2009).
Atelectrauma per se is deleterious, as demonstrated by Muscedere who showed, in a model of ventilated isolated lavaged lungs, that ventilation with PEEP below the inflection point increased lung injury (Muscedere et al. 1994). Moreover, lung collapse implies the use of higher peak pressures and larger tidal volumes in order to reexpand the lung, leading to a synergistic increase of lung injury. Lung recruitment is therefore a crucial element to minimize lung injury.
33.2.2.2 Oxygen Therapy
It has been known for a long time that BPD is closely linked to oxygen therapy. The mechanisms of oxygen injury have been discussed in detail elsewhere (Maltepe and Saugstad 2009). Hyperoxia is a widely used model of arrested alveolar development in rodents (Randell et al. 1989) and can be used to study the disruption of the normal factors of alveolar development. For example, rat pups exposed to hyperoxia exhibit disruption of elastic fibers (Bruce et al. 1989) and elastin synthesis and assembly (Bland et al. 2007b) and a decrease in alveolar cell expression of vascular endothelial growth factor (Lopez et al. 2006; Maniscalco et al. 1997) or several other growth factors (Bland et al. 2007a; Wagenaar et al. 2004). The effect of oxygen may increase events that occurred antenatally, namely, prenatal inflammation (Choi et al. 2009) and induce inflammation as described below.
33.2.2.3 Inflammation
Finally, and closely linked to the previous factors, inflammation is one of the prominent mechanisms of lung injury. It results from treatments such as oxygen, leading to the generation of reactive oxygen species. It also results from volutrauma and atelectrauma that, along with reactive oxygen species and local inflammation or bacterial colonization, induce an inflammatory response that plays a major role in the pathogenesis of BPD. This inflammation may be self-perpetuating, accounting for residual damage leading to chronic lung injury.
Increased concentrations of proinflammatory cytokines have been detected in the amniotic fluid and in tracheal aspirate within hours after birth in newborns who subsequently developed BPD (Speer 2009). Thus, “fetal inflammatory response syndrome” is usually considered to be a causative factor of BPD, although this factor is more controversial in the clinical setting (Kent and Dahlstrom 2004; Richardson et al. 2006). BPD is associated with an inflammatory response of the airways characterized by the presence of neutrophils and activated macrophages, high concentrations of proinflammatory cytokines and chemokines (IL-8, TNF-α, IL-1, IL-6), and increased expression of ICAM adhesion molecules and L-selectin that trigger the recruitment of circulating neutrophils (Speer 2006, 2009). Large numbers of activated neutrophils invade the airspaces within hours after birth and persist during the first week of life in the airways of infants with BPD (Merritt et al. 1983). Further lines of evidence suggest that neutrophils of preterm infants who develop BPD are less prone to undergo apoptosis (Hanna et al. 2005; Oei et al. 2003). Blood cytokines have been shown to be elevated in infants evolving toward BPD or death (Ambalavanan et al. 2009). Experimental induction of a pulmonary inflammatory response in neonatal animals is associated with disorders of alveolar development (Franco et al. 2002). Neutrophil influx, which is triggered by mechanical ventilation in preterm animals (Carlton et al. 1997), plays a crucial role. Rat pups treated with antibodies to the neutrophil chemokine cytokine-induced neutrophil chemoattractant-1 (CINC-1) during 95 % O2 exposure present reduced adverse effects of hyperoxia-induced inflammation on lung development (Auten et al. 2001b). Many iatrogenic factors such as mechanical ventilation (Ricard and Dreyfuss 2001; Tremblay et al. 2002) or oxygen therapy are able to induce an inflammatory response as well as bacterial infections (Akram Khan et al. 2006; Ehrenkranz et al. 2005; Maxwell et al. 2009).
Inflammation, presence of reactive oxygen species, and disturbance of normal biological mechanisms of alveolar development can account for what is called “biotrauma.”
33.2.2.4 Immediate Consequences of Lung Injury
The main consequences of ventilator-induced lung injury (VILI) can be summarized as (Auten et al. 2001a; Miller and Carlo 2008):
-
Alveolar capillary leak: high tidal volumes result in the disruption of alveolar and airway epithelium, basement membranes, and pulmonary capillary endothelium, a process which leads to fluid and protein leaks and exacerbates respiratory failure.
-
Surfactant inactivation by proteins and serum components which add to surfactant deficiency if present, thereby increasing surface tension and worsening capillary leaks.
-
Inflammation: mechanical ventilation increases accumulation of inflammatory cells and mediators in the lung. In experimental studies, the greatest increase in proinflammatory cytokines is linked with high inflation pressures and high tidal volume.
-
Air leaks: the mechanisms of which are discussed in another chapter, particularly, pulmonary interstitial emphysema which is an important factor of progression to BPD.
-
Distal organ effects linked to the systemic diffusion of inflammatory cells and soluble mediators.
33.2.3 Treatment Options for Lung Protection
Mechanical ventilation settings that can increase or decrease lung injury are therefore:
-
Oxygen therapy: oxygen must be considered as a potentially very harmful drug for the lung that is certainly involved in biotrauma, especially in preterm infants.
-
Continuous positive airway pressure (CPAP): introduced by Gregory in 1971 (Gregory et al. 1971), the use of CPAP (also termed PEEP) rapidly became a standard of ventilatory care. It is an essential means to limit atelectrauma, as it maintains FRC and consequently alveolar inflation during expiration. It has been proposed to combine PEEP with recruitment maneuver in order to reopen collapsed alveoli, also known as open lung ventilation (van Kaam and Rimensberger 2007).
-
Peak inspiratory pressure: it determines the tidal volume and is thus necessary in mechanical ventilation, but it may be responsible for barotrauma, volutrauma, and lung overexpansion.
-
Mechanical ventilation rate, inspiratory time, and inspiratory/expiratory ratio.
-
The resultant of these combined settings, mean airway pressure which may also be involved in neonatal lung injury (Greenspan et al. 2004).
Prevention of lung injury can therefore be summarized as (van Kaam and Rimensberger 2007) prevention of volutrauma by limiting tidal volumes, as performed in adults, and application of PEEP to maintain FRC and reopen collapsed alveoli by recruitment maneuvers. The various ventilation modes that can achieve these combined goals and their results on the main consequence of neonatal lung injury, bronchopulmonary dysplasia, are discussed below. Prevention of air leaks is discussed in another chapter.
33.2.4 Practical Measures to Reduce the Likelihood of VILI in Neonatal Patients, e.g., to Prevent BPD
33.2.4.1 Predisposing Factors
Bronchopulmonary dysplasia (BPD) is the main consequence of VILI in neonatal period. This disease first described by Northway at the end of the 1960s as the major form of respiratory sequelae observed in preterm infants (Northway et al. 1967). The main clinical characteristic of this disease is the need for prolonged supplemental oxygen in preterm infants. This oxygen dependence constitutes the common denominator of the various definitions of BPD proposed since its first description. Two evaluation periods have been proposed: 28 days after birth and at the corrected age of 36 weeks of gestation. Evaluation at 36 weeks of gestation was very rapidly found to be more closely correlated with medium-term and long-term respiratory outcome than the evaluation at 28 days (Ehrenkranz et al. 2005; Shennan et al. 1988).
BPD is essentially a disease of very preterm infants. In Europe, the mean BPD rate at 36 weeks of gestation is 15.8 % in preterm infants born before 32 weeks, with variations of 10.5–21.8 % between countries (Zeitlin et al. 2008). Data from the National Institute of Child Health and Human Development Neonatal Research Network show a mean BPD rate at 36 weeks of 22 % in preterm infants with a birth weight less than 1,500 g (Fanaroff et al. 2007). The BPD rate is closely correlated with the degree of prematurity. At a gestational age of 36 weeks, 46 % of preterm infants with a birth weight less than 750 g have BPD compared to 33 % of infants with a birth weight between 750 and 1,000 g and 10 % of those with a birth weight between 1,000 and 1,500 g (Fanaroff et al. 2007).
Several parameters related to the infant itself and its environment have been identified as independent risk factors for subsequent development of BPD. Independently of inflammatory and infectious factors, the duration of postnatal exposure to oxygen and the duration of mechanical ventilation are very significantly correlated with the risk of subsequent BPD (Akram Khan et al. 2006). Hemodynamic factors also play a predisposing role, such as persistence of a patent ductus arteriosus (Akram Khan et al. 2006) or the need for repeated plasma expansion (Korhonen et al. 1999; Oh et al. 2005).
However, the major risk factor is host immaturity, as the incidence of BPD increases very significantly with the degree of prematurity (Akram Khan et al. 2006; Ehrenkranz et al. 2005). Moreover, small gestational age infants have an increased risk of BPD (Ehrenkranz et al. 2005; Korhonen et al. 1999). Genetic susceptibility factors have also been proposed (Bhandari et al. 2006; Lavoie et al. 2008).
The first descriptions of BPD generally referred to infants with respiratory distress syndrome (RDS) mechanically ventilated with supplemental oxygen, and the prevention of BPD was initially focused on these aspects: prevention and treatment of RDS, improvement of mechanical ventilation, and control of oxygen therapy, which remain the objectives of respiratory support in NICU.
33.2.4.2 Prevention of Mechanical Ventilation-Induced Lung Injury
33.2.4.2.1 Antenatal Steroid Therapy
Steroids constitute an essential treatment during threatened preterm delivery, and the efficacy of this treatment for the prevention of RDS has been very largely demonstrated. As they limit the need for mechanical ventilation, it could have been expected an effect on lung injury. In fact, data concerning the efficacy of steroids on BPD are contradictory (Van Marter et al. 1990, 2001), but, globally, meta-analyses do not clearly demonstrate any beneficial prophylactic effect on BPD (Roberts and Dalziel 2006) regardless of the type of steroid used (Brownfoot et al. 2013).
33.2.4.2.2 Respiratory Support in the Delivery Room
Improved management in the delivery room is probably one of the main factors of prevention of the development of BPD, as lung lesions can be induced in the first minutes of life (Jobe et al. 2008). The harmful effect of large tidal volumes immediately after birth has been extensively studied experimentally (Hillman et al. 2007; Ikegami et al. 2000). The choice of prolonged inspiration rather than large tidal volume ventilation has been proposed to establish FRC. A randomized trial compared the use of prolonged high pressure inspiration followed by early initiation of nasal CPAP vs. conventional management consisting of mask ventilation and initiation of nasal CPAP in the intensive care unit. The first strategy allowed a reduction of the BPD rate (te Pas and Walther 2007), confirming the importance of limiting the tidal volume during the first minutes of life and the value of prolonged insufflation to establish FRC. Current ILCOR guidelines recommend limiting insufflation pressures to less than 20 cm H2O (ILCOR 2006).
33.2.4.2.3 Exogenous Surfactants
Exogenous surfactants, by limiting the aggressiveness and duration of mechanical ventilation, raised great hopes for the prevention of BPD. The administration of exogenous surfactants in infants with RDS is effective to prevent the combined risk of death and BPD, but generally not the risk of BPD alone (Soll 2000, 2010). The efficacy of surfactants is more marked when they are administered early, within the first 2 h of life (Yost and Soll 2000), or prophylactically, e.g., immediately after birth (Soll and Morley 2001). The new peptide-containing artificial surfactants are now as effective as natural surfactant (Moya et al. 2005; Pfister et al. 2007; Sinha et al. 2005). Early administration of surfactant combined with rapid extubation could be a safe approach (Stevens et al. 2007). It has been proposed to maintain long-term surfactant therapy for prevention of BPD. A pilot trial of this strategy has been performed, but no definitive conclusions can be drawn, and this treatment therefore cannot be recommended at the present time (Laughon et al. 2009).
33.2.4.2.4 Ventilatory Modes
Several approaches have been proposed in order to achieve the goals defined in Sect. 33.2.2.5. Some of them will be discussed below, bearing in mind that new techniques will probably be evaluated over the next few years. The use of noninvasive ventilation (or less invasive ventilation) consisting of management by spontaneous breathing with continuous positive airway pressure can prevent or limit the progression of collapse of distal airspaces while avoiding systematic endotracheal intubation. This approach nevertheless requires intubation for administration of exogenous surfactant as prophylaxis or when the diagnosis of RDS is confirmed. Studies performed in the baboon model have demonstrated the efficacy of CPAP to prevent BPD (Thomson 2002; Thomson et al. 2004, 2006). The COIN trial, in which surfactant was not administered systematically, showed a reduction of the “oxygen at 28 days or death” endpoint in the CPAP arm, but did not demonstrate any effect on the “oxygen at 36 weeks or death” endpoint or on the need for oxygen therapy on discharge from hospital (Hascoet et al. 2008; Morley et al. 2008). Other ongoing or finished trials will bring new data to these one.
Nasal intermittent positive pressure ventilation (NIPPV), which can be an alternative to intubation, is discussed elsewhere, and further studies are required to assess its effect on BPD.
Synchronization of the neonate with the ventilator (use of the trigger) represents a definite progress in conventional ventilation, but no published study has demonstrated the value of synchronized ventilatory modes on the incidence of BPD (Greenough et al. 2008). At the very most, the study by Bernstein (Bernstein et al. 1996) reported a significant reduction of the proportion of infants with a birth weight below 1,000 g, oxygen-dependent at 36 weeks (72 % in intermittent mandatory ventilation vs. 47 % in synchronized intermittent mandatory ventilation), demonstrating the value of synchronization. Other ventilatory modes can be proposed, but their efficacy for the prevention of BPD has not yet been demonstrated. Volume-targeted ventilation, usually not used in the neonatal period has been more studied recently. It is designed to limit volutrauma, which can be achieved in two ways: volume-targeted ventilation in which pressure is controlled and volume-controlled ventilation in which a specific tidal volume is delivered by the ventilator irrespective of the state of lung compliance or the pressure required. Long-term respiratory outcome has been less thoroughly studied with these modes, and it is not possible to conclude on their effect on BPD (Grover and Field 2008; McCallion et al. 2005; Sinha and Donn 2008).
More than 10 years after the first trials of early use of high-frequency oscillation (HFO) ventilation in very preterm infants with RDS, it has still not been clearly established whether this technique is able to reduce the rate of long-term respiratory sequelae. The most recent trials compared the results of HFO and conventional mechanical ventilation in 2001 and 2002. The trial performed in France studied infants under the age of 30 weeks with RDS (Moriette et al. 2001). No significant difference was demonstrated in terms of the incidence of BPD. The trial performed in the United Kingdom confirmed this result (Johnson et al. 2002). Only the American study demonstrated a very limited benefit on the BPD rate (Courtney et al. 2002). The Cochrane meta-analysis on this subject indicates a beneficial but limited result (Henderson-Smart et al. 2009), but a careful review of the meta-analysis shows that several parameters were not taken into account which precludes definitive conclusions on this point (van Kaam and Rimensberger 2007).
Permissive hypercapnia, widely proposed in adults for a number of years, can reduce barotrauma and volutrauma, which tend to worsen lung injury. Few randomized trials have been performed and have failed to demonstrate any effect on the incidence of BPD (Woodgate and Davies 2001).
33.2.4.3 Oxygen Administration
Oxygen is a well-known risk factor for subsequent BPD. Initial resuscitation of asphyxic term infants with room air is as effective as and less harmful than resuscitation with pure oxygen (Saugstad et al. 2008). It is difficult to extrapolate these data to preterm infants which have been less studied (Vento et al. 2009), but, in view of its potential toxicity, the use of oxygen must be closely monitored right from the delivery room.
33.2.4.4 Anti-inflammatory Agents
33.2.4.4.1 Nonsteroidal Anti-inflammatory Therapy
Pentoxifylline is a phosphodiesterase inhibitor possessing anti-inflammatory properties. It was used in a recent trial of nebulization and appeared to decrease the incidence of BPD. However, this treatment is still at a very preliminary phase of development (Lauterbach et al. 2006).
33.2.4.4.2 Steroid Therapy
As the inflammatory reaction of the lung is probably one of the major factors leading to BPD, it was logical to use corticosteroids, which are powerful anti-inflammatory agents. Three types of steroid regimens were used for at least 20 years: (1) very early treatment during the first 96 h of life followed by rapid reduction; (2) moderately early treatment between the 7th and 14th days of life; and (3) late treatment after the 3rd week of life, generally in infants with persistent ventilatory assistance due to severe BPD. It should be noted that these protocols conducted in the early 1990s very often concerned less preterm populations than those essentially concerned by BPD, with a very low antenatal steroid therapy rate and without the use of surfactant in some trials. Postnatal dexamethasone reduces mechanical ventilation requirements at 28 days of life and at 36 weeks of corrected age. However, early or moderately early steroid therapy is not accompanied by a reduction of oxygen dependence at term, and none of the three treatment regimens is associated with a reduction of neonatal mortality (Halliday et al. 2003a, b, c). Two more recent meta-analyses of administration of steroids before or after 7 days emphasize that the long-term neurological risks are essentially associated with early steroid therapy, but that the risk of severe retinopathy persists for steroids administered after the first week of life (Halliday et al. 2009a, b).
An attractive approach is that proposed by K. Watterberg for the most preterm infants, which consists of early administration of very low doses of hydrocortisone hemisuccinate designed to prevent the adrenal insufficiency observed at these gestational ages. Unlike the pilot study (Watterberg et al. 1999), the multicenter trial did not demonstrate any efficacy on BPD except in infants born in a context of chorioamnionitis, and this trial had to be interrupted due to an excess of gastrointestinal perforations attributed to the combined use of indomethacin (Watterberg et al. 2004).
An alternative would be the use of inhaled steroids, although they have not been clearly demonstrated to be effective for the prevention of BPD. However, inhaled steroids decrease the duration of mechanical ventilation and reduce the subsequent need for systemic steroids (Shah et al. 2012). Although no serious adverse effects have yet been attributed to inhaled steroids, they must be used very cautiously in view of the experience acquired with systemic steroids.
33.2.4.5 Other Proposed Preventive Treatments
33.2.4.5.1 Nitric Oxide
Apart from its recognized efficacy for the treatment of persistent pulmonary artery hypertension in neonates, NO is one of the promising strategies for prevention of bronchopulmonary dysplasia (BPD). This choice is based on well-documented pathophysiological mechanisms, especially abnormalities of pulmonary microvascular development during pathological alveolarization and the role of NO in these phenomena. Several experimental studies have provided positive results on alveolarization (Lin et al. 2005; Maniscalco et al. 2002; McCurnin et al. 2005; ter Horst et al. 2007). Finally, several large-scale randomized trials have been published with contradictory results, making it difficult to reach any final conclusions. The meta-analysis on this subject concluded that the use of NO for the prevention of BPD is not currently recommended but could be considered in certain subgroups (Barrington and Finer 2007a, b). Nevertheless, a recent review of these results (Kinsella and Abman 2007) suggests that the effects of inhaled NO are dependent on the time of administration, the dose used, the duration of treatment, the underlying disease and its severity, and the population.
The effects of NO on laboratory parameters and pulmonary function tests were studied in parallel in the clinical trial conducted by Ballard (Ballard et al. 2006). This study demonstrated that NO did not modify the protein and phospholipid composition of surfactant and improved the surfactant properties of tracheal aspirate fluid (Ballard et al. 2007) without modifying markers of inflammation (Truog et al. 2007) and without increasing plasma markers of oxidative stress (Ballard et al. 2008).
A possible treatment for the future could be the administration of angiogenic factors such as VEGF in order to improve pulmonary vasculature (Thebaud et al. 2005).
33.2.4.5.2 Closure of Ductus Arteriosus
Although patent ductus arteriosus is probably associated with BPD (Bancalari et al. 2005), treatment of patent ductus arteriosus, either medical with nonsteroidal anti-inflammatory drugs such as indomethacin or ibuprofen (Ohlsson et al. 2010) or surgical, has not been clearly demonstrated to be effective in the prevention of BPD. In an experimental model in the baboon, a beneficial effect of ibuprofen therapy was demonstrated on alveolarization (McCurnin et al. 2008), while no such effect was observed after surgical ligation (Chang et al. 2008). However, no clear data are available in human preterm infants (Mosalli and Alfaleh 2008; Raval et al. 2007). Fine characterization of the ductus arteriosus will probably be necessary to predict the efficacy of treatment (McNamara and Sehgal 2007), especially as some authors consider that conservative management comprising fluid restriction and adjustment of ventilation may be sufficient to obtain spontaneous closure of patent ductus arteriosus (Vanhaesebrouck et al. 2007).
33.2.4.5.3 Fluid Restriction and Diuretics
Although the association between BPD and high fluid intake has been reported on several occasions, the efficacy of moderate fluid restriction for prevention of BPD has not been demonstrated, although the meta-analysis on the subject reported a tendency toward reduction (Bell and Acarregui 2008). Diuretics, which improve pulmonary function in dysplastic infants, have also not been shown to be effective when administered early to prevent BPD (Brion and Soll 2008).
33.2.4.5.4 Vitamin A
Retinoic acid is essential in the control of septation (Bourbon et al. 2005), and hyperoxic rat pups treated by retinoic acid may recover normal alveolarization. Vitamin A supplementation has been formally demonstrated to be effective in the prevention of BPD. The Cochrane meta-analysis showed a limited but real benefit in terms of BPD at 28 days and 36 weeks of corrected age, suggesting the value of optimal vitamin A supplementation (Darlow and Graham 2011).
33.2.4.5.5 Caffeine
Caffeine is probably one of the most frequently prescribed treatments in preterm neonates, essentially for the prevention of central apneas. A large-scale international multicenter randomized placebo-controlled study of caffeine included 2,000 infants with a birth weight between 500 and 1,250 g treated with either caffeine citrate or placebo. In the short term (Schmidt et al. 2006), treatment with caffeine was associated with a significant reduction of the duration of tracheal ventilation, noninvasive ventilation, and oxygen therapy (each reduced by about one week). The respiratory benefit was observed on the BPD rate defined at 36 weeks of postmenstrual age (36.3 % in the caffeine group vs. 46.9 % in the placebo group).
33.2.5 Conclusion
Neonatal lung injury leads to BPD which is its main respiratory complication. However, this disease is now dominated more by immaturity than by the consequences of mechanical ventilation. It is therefore very difficult to show that a single and very specific intervention can have a positive effect, which is why, in contrast with clinical experience, some treatments are not found to be effective in randomized trials. Overall management therefore needs to be evaluated globally; a recent study clearly demonstrated the efficacy of multiple interventions (Geary et al. 2008).
Essentials to Remember
-
Use quickly optimal CPAP to maintain FRC.
-
Limit tidal volumes.
-
Limit oxygen delivery.
-
Administer surfactant as soon as possible.
-
Pay attention to other factors such as nutrition and other preventive agents.
33.3 Ventilator-Associated Lung Injury in the PICU
Thomas Jaecklin6 and Brian P. Kavanagh6
(6)
Departments of Anesthesia and Critical Care Medicine, Hospital for Sick Children, University of Toronto, 555 University Avenue, Toronto, ON, M5G 1X8, Canada
Educational Goals
In this chapter, the reader can learn:
-
The definition of ventilator-associated lung injury (VALI), its clinical interface with the acute respiratory distress syndrome (ARDS) and ventilator-induced lung injury (VILI), and its epidemiology
-
The main mechanisms by which mechanical ventilation can injure the lung
-
The etiology and clinical presentation of ARDS and VALI
-
The most frequently used methods to reduce the impact of mechanical ventilation on the lung
33.3.1 Mechanisms of VILI
Many different mechanisms of VILI have been described (Fig. 33.1) and will be discussed with a focus on pediatric patients.
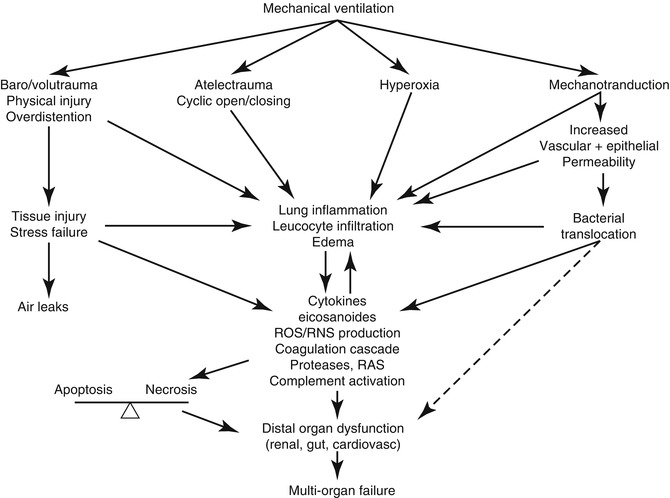
Fig. 33.1
Mechanisms of ventilator-induced lung injury and consequences on other end organs. ROS/RNS reactive oxygen/nitrogen species, RAS renin-angiotensin system
33.3.1.1 Physical Injury
33.3.1.1.1 Baro- and Volutrauma
Initially, air leaks (i.e., accumulation of extra-alveolar air either in the interstitium, mediastinum, pleural cavity, or even the subcutaneous tissue) were the key recognized adverse effect of mechanical ventilation (Pierson 2006). This was thought to be the consequence of mechanical disruption of airspace integrity due to excessive airway pressure (barotrauma). Soon it was observed that large V T is responsible for the VILI rather than high airway pressures.
To distinguish whether pressure or volume was the injurious agent, a series of studies were undertaken. Rats (Dreyfuss et al. 1988), rabbits (Hernandez et al. 1989), or lambs (Carlton et al. 1990) with healthy lungs were ventilated with either high or low V T, but the peak inspiratory pressures were kept the same by limiting inspiratory thoracoabdominal excursions with straps or casts. In contrast to the animals ventilated with low V T, those ventilated with high V T developed pathological and ultrastructural changes of VILI. These ultrastructural changes have been well characterized as tears in the pulmonary endothelium and epithelium due to cellular stress failure (Fu et al. 1992). Whether these lesions at the cellular level are the actual cause of the air leak that can be observed clinically is not established, but the extrapolation is compelling. Nonetheless, these experiments made a strong case that volume, and not pressure, was the main culprit (volutrauma).
The hypothesis that high V T ventilation is the major contributor to VILI is supported by the findings of a large randomized controlled trial in adults suffering from ARDS, where the mortality was higher in patients ventilated with a V T of 12 vs. 6 mL/kg (ARDS Network 2000).
33.3.1.1.2 Atelectrauma
Not only high V T can induce or worsen lung injury. Mechanical ventilation with low V T in the absence of PEEP can also lead to lung injury. This was believed to result from cyclic opening and closing (recruitment and de-recruitment) of terminal airspaces (Chu et al. 2004) that lead to lung inflammation and diffuse alveolar damage and was termed atelectrauma. Another possible explanation for this finding was suggested by Mead et al. (1970). Using a mechanical model of interdependent alveoli, he showed that despite a homogeneous transalveolar pressure of 30 cm H2O, the walls of collapsed alveoli could experience much higher pressures (up to 140 cm H2O) due to this interdependence. However, in at least one experimental model, the injury in atelectatic areas is minimal, in contrast to that in the aerated (i.e., nonatelectatic zones) (Tsuchida et al. 2006); in this sense, atelectasis may cause injury by exacerbating the stress in the residual aerated lung.
33.3.1.1.3 Oxygen Toxicity
Another mechanism of physical injury to the lung during mechanical ventilation results from oxygen toxicity. High inspired O2 concentrations are often required in patients with severe respiratory failure to maintain adequate blood O2 levels. Nash et al. mechanically ventilated goats with low airway pressures and either room air or 100 % fraction of inspired oxygen (Nash et al. 1971). The hyperoxic animals did not survive more than 4 days on the ventilator, and their lungs showed pathological changes as described above; in contrast, animals breathing room air remained well for up to 2 weeks, and their lung histology was normal. The O2 toxicity is thought to be mediated through the harmful effect of ROS and peroxidized membrane lipids. Both are produced in excess in the presence of high levels of inspired O2 (Weinberger et al. 2002). Oxygen levels of 60 % or higher have been consistently shown to induce oxidative stress resulting in inflammation, decreased lung cell proliferation, and altered respiratory mechanics. Especially in children, the histological image is similar to bronchopulmonary dysplasia. The underlying cellular mechanisms for this finding include dysregulated inflammation, altered protease/antiprotease balance, and altered growth or differentiation of lung cells either due to a direct effect of O2 or due to the harmful compounds mentioned above.
33.3.1.2 Inflammatory Response to Stretch: The Biotrauma Hypothesis
After focusing on the physical effects of mechanical ventilation, it was progressively realized that VILI is an inflammatory disease, largely dependent on neutrophils (Kawano et al. 1987). The exact mechanism by which mechanical ventilation induces this inflammatory process is not clear, but a contemporary theory is the so-called biotrauma hypothesis (Tremblay and Slutsky 1998), which suggests that mechanical stretch induces the production of inflammatory mediators that contribute to the lung injury and ultimately might lead to multiorgan failure, the primary cause of mortality in patients with severe respiratory failure.
33.3.1.2.1 Inflammatory Cytokines
Evidence supporting the biotrauma hypothesis is largely based on circulating or pulmonary cytokines. It can be expressed as follows: Injurious mechanical ventilation, i.e., high V T and/or low PEEP, is associated with higher concentrations of inflammatory mediators in human studies (ARDS Network 2000; Ranieri et al. 1999; Stuber et al. 2002) and induces their production in experimental models of VILI in vivo (Wilson et al. 2003) and ex vivo (Tremblay et al. 1997; von Bethmann et al. 1998; Yoshikawa et al. 2004). Cyclic cell stretch in vitro also leads to secretion of cytokines and chemokines (Pugin et al. 1998; Vlahakis et al. 1999). Interventions directed against the production or actions of proinflammatory mediators have been shown to decrease the degree of VILI in experimental studies. Glucocorticosteroids appear to block the proinflammatory nuclear factor κB (NF-κB), one of the main proinflammatory transcription factors, and decrease both the level of cytokine mRNA transcription and the degree of lung injury (Held et al. 2001). Several anti-cytokine strategies have led to an improvement of markers of VILI: intratracheal application of polyclonal antitumor necrosis factor-α (TNF-α) antibodies attenuated the impairment of lung compliance, improved histological abnormalities, and reduced leukocyte infiltration after high V T ventilation (Imai et al. 1999). Systemic anti-macrophage inflammation protein-2 (MIP-2) antibodies reduced the leukocyte migration induced by hyperoxia/hyperventilation (Quinn et al. 2002). Finally, recombinant interleukin-1 (IL-1) receptor antagonists decreased lung injury and leukocyte infiltration (Narimanbekov and Rozycki 1995).
It is important to recognize that causation has not yet been proved in any of the forgoing. As well, some of the experimental data suggest that the link between proinflammatory mediators and VILI might not be a simple “cause and effect” relationship. For example, some groups were unable to detect increases in inflammatory mediators, in particular TNF-α, using in vivo and in vitro models of VILI (Haitsma et al. 2000; Ricard et al. 2001; Verbrugge et al. 1999). Quinn et al. documented unchanged chemokine concentrations and neutrophil recruitment despite the induction of significant lung edema after 2 h in a rat model of high V T injury (Quinn et al. 2002). Also the administration of aerosolized recombinant TNF-α and interferon-γ to spontaneously breathing rats modulated the function of pulmonary and systemic monocytes, but led to only minimal pulmonary edema and inflammation (Debs et al. 1988), suggesting that these mediators are not pathogenic per se. Finally, Wrigge et al. ventilated 39 healthy patients scheduled for elective surgery under general anesthesia during 1 h with parameters that are generally considered injurious (V T 15 mL/kg without PEEP) vs. a so-called protective ventilation strategy (V T 6 mL/kg, PEEP of 10 cm H2O) and detected no difference in plasma cytokine levels between the two groups (Wrigge et al. 2000). Finally, mediators other than cytokines have also been investigated as potentially pathogenic in the context of VILI.
33.3.1.2.2 Coagulation Factors
Intra-alveolar fibrin is common in injured lungs, and this observation has preceded the discovery of “cross talk” among inflammatory mediators and the coagulation cascade during lung injury (Schultz et al. 2006). Proinflammatory mediators can stimulate the expression of tissue factor, thereby activating coagulation (van der Poll et al. 2001), and can suppress activation of protein C and promote the secretion of thrombomodulin and plasminogen activator inhibitor-1 (PAI-1). Increased levels of thrombomodulin and PAI-1, with reciprocal decreases in activated protein C (APC), exist in patients with ARDS and correlate with outcome (Ware et al. 2006). Finally, injurious ventilation is associated with similar perturbations (Dahlem et al. 2005), whereas protective ventilation may attenuate coagulation activation and prevent inhibition of fibrinolysis (Ware et al. 2006; Choi et al. 2006).
Because of these associations, components of the coagulation system have been identified as potential therapeutic targets. Heparin, initially promising because of anti-inflammatory as well as anticoagulant properties (Hochart et al. 2006), has little net benefit in the clinical setting (MacLaren and Stringer 2007). Other interventions have been disappointing also: Tissue factor pathway inhibitor (tifacogin) did not attenuate lung injury in a clinical trial (Abraham et al. 2003), and APC – does not improve outcome in patients with lung injury (Liu et al. 2008) despite its anti-inflammatory, antiapoptotic, and anticoagulation activity. Finally, thrombomodulin, an important cofactor in fibrin formation and activation of protein C, has a complex relationship with lung injury and is being evaluated as a potential therapeutic target (MacLaren and Stringer 2007; Ware et al. 2003).
33.3.1.2.3 Hormones
A prototypic hormone related to lung injury is angiotensin II. This hormone increases tissue vascular permeability via the production of eicosanoids and vascular endothelial growth factor as well as by alteration of cytoskeletal proteins (Suzuki et al. 2003). In addition, by enhancing intercellular adhesion and chemokine expression, angiotensin II augments migration of inflammatory cells and promotes tissue repair (Suzuki et al. 2003). Several effects point to a potential role in VILI. First, high V T upregulates important components of the renin-angiotensin system and increases expression of angiotensin receptors (Jerng et al. 2007). Second, the genotype (i.e., DD) coding for more active angiotensin-converting enzyme (ACE) is more prevalent in critically ill patients who have ARDS (vs. those who do not); and, among those with ARDS, the DD genotype is associated with higher mortality (Marshall et al. 2002). Third, pretreatment with the ACE inhibitor captopril (or the angiotensin II receptor antagonist, losartan) attenuates VILI in vivo (Jerng et al. 2007). Finally, ACE-2 is a captopril-insensitive ACE homolog that inactivates angiotensin II; it protects against experimental lung injury (Imai et al. 2005). Thus, circulating angiotensin II represents a candidate mediator. However, research continues to better understand the complex renin-angiotensin system. For example, several angiotensin II receptors have been described, and while one receptor type (AT1a) promotes lung injury, another (AT2) appears to protect (Imai et al. 2005).
33.3.1.2.4 Lipid-Derived Mediators
Levels of eicosanoids and platelet-activating factor (PAF) correlate with lung injury in several animal models of VILI (Goggel and Uhlig 2005; Miyahara et al. 2008). These molecules are potent modulators of vascular tone, permeability, inflammation, and platelet activation. They form a complex network, however, and may have proinflammatory or anti-inflammatory activity depending on the context. Inhibition of the proximal regulatory enzyme (cytosolic phospholipase A2; cPLA2) attenuates capillary permeability induced by high V T (Miyahara et al. 2008); and inhibition of all downstream enzymes (i.e., cyclooxygenase, lipoxygenase, and cytochrome P450) is required for effective protection, suggesting complex overlapping function (Miyahara et al. 2008). Another important candidate lipid mediator is PAF, which increases platelet activation, leukocyte attraction, and microvascular permeability, in part via increasing intracellular Ca2+, and in part via synthesis of prostaglandins and ceramides (Goggel et al. 2002). Earlier preclinical inhibition of the PAF receptor was effective (Nagase et al. 1999), but clinical trials did not demonstrate an impact on mortality (Johnson et al. 2001). Ceramides (and other sphingolipids, e.g., sphingosine-1-phosphate and sphingomyelin) have recently been recognized as potentially important in VILI (Uhlig and Gulbins 2008), regulating apoptosis and integrity of intercellular junctions. Increased acid sphingomyelinase activity (resulting in elevated ceramide levels) occurs in the lungs of patients with ARDS (Rauvala and Hallman 1984), and in sepsis, the circulating levels correlate with mortality (Claus et al. 2005; Drobnik et al. 2003). Finally, inhibition of acid sphingomyelinase stabilizes surfactant (von Bismarck et al. 2008) and attenuates pulmonary inflammation in in vivo lung injury (Uhlig and Gulbins 2008).
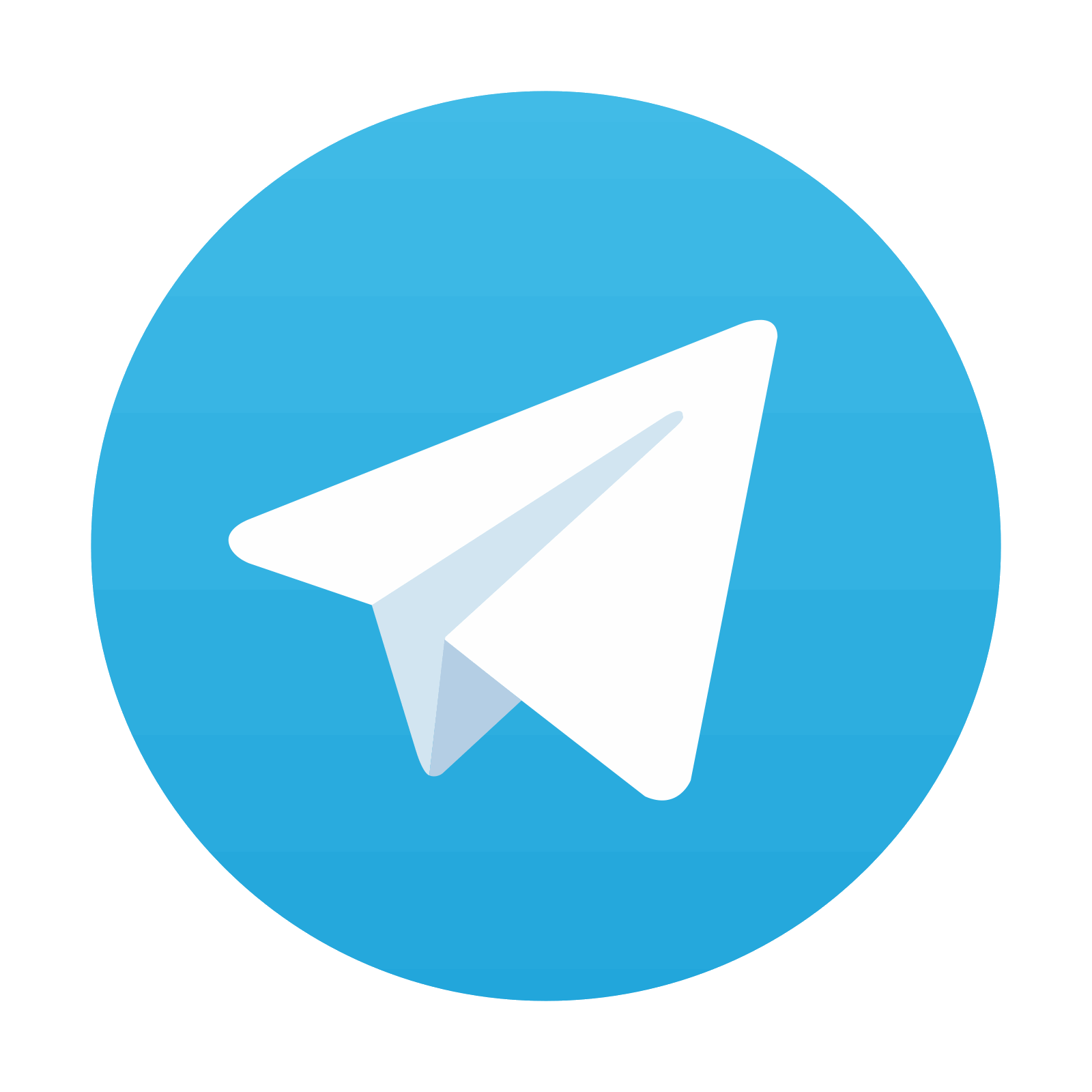
Stay updated, free articles. Join our Telegram channel
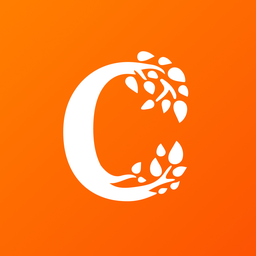
Full access? Get Clinical Tree
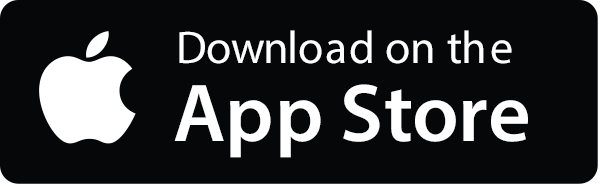
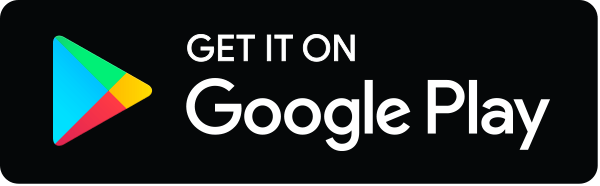