Thyroid hormone is critical for linear growth and for maturation of thyroid-dependent tissues, including the brain. Many physiologic factors influence fetal and neonatal thyroid function. They include embryogenesis of the thyroid, fetal-maternal relationships, and the dynamic alteration of thyroid function with birth, the action of thyroid hormones, the synthesis and transport of these hormones, and mechanisms regulating thyroid function. Thyroid hormone abbreviations are defined in Box 97-1. To provide the background necessary to understand thyroid disorders in infants, this chapter begins with sections on thyroid physiology and laboratory tests. Embryology and fetal development of thyroid function are then described. Finally, clinical conditions of altered thyroid function are discussed. The principal functions of the thyroid gland are to synthesize, store, and release the thyroid hormones thyroxine (T4) and triiodothyronine (T3) into the circulation. The major secretory product of the thyroid is T4. Thyroidal secretion of T3 accounts for only about 20% of its production. The remaining 80% is derived from peripheral deiodination of T4. Therefore, T4 acts as a prohormone for T3 because T4 has negligible intrinsic metabolic activity in most tissues. Most of the physiologic effects of thyroid hormone are mediated by T3 through its interaction with the thyroid response element on DNA.4 Congenital thyroid hormone deficiency results in cretinism (severe mental retardation) if not treated. This effect is prevented by thyroid hormone replacement early in life. Newborn screening for thyroid deficiency was initiated in Quebec in 1974. Intellectual development is normal in children with congenital hypothyroidism who are treated early and aggressively and is significantly better in those treated early (by 7 days of age) and with higher thyroid hormone doses (12 µg/kg per day) than in those started on therapy later or treated with lower doses.13 Prenatal and postnatal maturation of the brain, retina, and cochlea is thyroid hormone dependent.37 Thyroid hormone modulates expression of thyroid hormone–responsive target genes at precise times during development, controlled by an interplay of deiodinases, thyroid receptor expression, transporters, cofactors, and transcription factors.4,12,33,89 T3 promotes the neural differentiation of embryonic stem cells at a dendritic level.14 T3 and T4 have an important impact on vascular development in the brain.98 Genes involved in neural migration are also regulated by thyroid hormone.12 Animal models have been developed for further study of thyroid effects on brain development.6,71 One of the principal actions of thyroid hormone is to stimulate rate of cellular oxidation in a large variety of tissues, leading to increased oxygen consumption, liberation of carbon dioxide, and production of heat. In hypothyroidism, the basal metabolic rate is reduced, but in hyperthyroidism, it is increased. The action of thyroid hormone may be mediated through increased protein synthesis. T3 binds to the thyroid response element in the cell nucleus, leading to enhancement of polymerase activity, which in turn leads to increased cellular messenger RNA (mRNA) and corresponding proteins.4 Synthesis of membranous sodium-potassium adenosine triphosphatase (ATPase) is enhanced by this mechanism. A large portion of oxygen consumption depends on sodium pump activity. T3 has direct effects on the mitochondria in vitro and probably in vivo.96 T4 in cell culture induces phosphorylation of mitogen-activated protein kinase. Thyroid hormone enhances response of beta-receptors to catecholamines without increasing the number of receptors. Effects of thyroid hormone on lipid metabolism are seen in the increased serum total cholesterol and low-density lipoprotein (LDL) cholesterol concentrations in hypothyroidism. The total neutral fats, fatty acids, apolipoprotein B, and phospholipids of the serum are also increased in hypothyroidism.43 A principal action of thyroid hormone is its effect on growth and development. These effects may be tissue specific and synergistic with other hormones. Prenatal growth is highly dependent on nutrition and insulin secretion. Postnatal linear growth is dependent on thyroid hormone and growth hormone (GH), which are mediated through insulin-like growth factor type 1 and its receptor. Similar synergistic effects between thyroid hormone and growth hormone can be observed in skeletal maturation. Thyroid hormone exerts its effects together with multiple hormones and growth factors. When primary hypothyroidism occurs, dental eruption, linear growth, and skeletal maturation are retarded. Retardation of skeletal maturation may be severe and is associated with immature skeletal proportions and facial contours, which contribute to the characteristic body configuration of hypothyroidism (long torso compared to short length of arms and legs) that is different from that seen in stunted growth caused by isolated GH deficiency. Ossification of cartilage is also disturbed in hypothyroidism, leading to epiphyseal dysgenesis in radiographs of the ossifying epiphyseal centers (Figure 97-1). Growth rate and adult height are normal in children with congenital hypothyroidism in whom thyroid hormone therapy is consistently maintained.61 This iodide-trapping mechanism involves an active transport process (an iodide pump [iodide symporter]) that requires oxidative phosphorylation. The iodide pump is a major rate-limiting step in thyroid hormone biosynthesis, and when it is defective, it is a rare cause of congenital goitrous hypothyroidism.25 The iodide pump is present at both the basal and apical surfaces of follicular cells. At the basal cell surface, the pump concentrates iodide in the cells by transporting them from the extracellular space. At the apical cell surface, the pump pushes iodide into the follicular lumen as a secondary reservoir. The mechanism is capable of maintaining intrathyroidal iodide concentration at a 20- to 100-fold higher level than that of serum. Some anions—bromide (Br2−), nitrate (NO22−), thiocyanate (SCN2−), perchlorate (ClO42−), and technetium pertechnetate (TcO42−)—are capable of competitively inhibiting iodide transport. Iodide is immediately oxidized to an active form for iodination of thyroglobulin (TG) by a peroxidase enzyme system.25 Thyroglobulin, a glycoprotein, is synthesized by the ribosomes of the follicular cells. Iodination of TG (organification) appears to occur at the cell colloid-lumen interface. Almost all the iodine taken up by the thyroid is rapidly incorporated into the 3 and the 5 positions of the many tyrosyl residues of TG to form monoiodothyronine (MIT) and diiodothyronine (DIT). Once it is organically bound to tyrosyl residues, iodine can no longer be readily released from the thyroid. Defects in iodide oxidation or organification can be seen in several types of goitrous cretinism. Secretion of T4 and T3 into the circulation requires the liberation of these moieties from TG.80 Thyroglobulin molecules pass from the lumen of the follicles into the follicular cells (endocytosis), where colloid droplets are ingested by lysosomes and undergo proteolysis. Congenital hypothyroidism may result from abnormal TG synthesis or metabolism. Of the about 125 tyrosyl residues in TG, only about 10 form iodothyronines, and another 20 consist of MIT and DIT. After proteolysis of TG, the freed MIT and DIT are deiodinated by iodotyrosine deiodinase, and the liberated iodide is recycled by the thyroid for reiodination of new TG. A defect in the deiodination mechanism of freed iodotyrosines results in depletion of iodine by release from the thyroid gland into the circulation and then excretion into the urine, resulting in goitrous cretinism. Neural tissue requires FT4 (and does not bind T3). Intracellularly, the FT4 is converted to FT3 by type II deiodinase. Type II deiodinase regulates T3 production from T4 in the pituitary, neuroglial cells, and astrocytes and is involved in the control of thyrotropin (TSH) secretion from the pituitary gland.77 Type III deiodinase, or 5-deiodinase, regulates peripheral deiodination of T4 at the 5 position on the thyronine molecule instead of 5′ and generates reverse T3 (rT3). Serum rT3 concentration parallels that of T3 in normal circumstances but not in fetal life, starvation, or patients with severe nonthyroidal illnesses (e.g., euthyroid sick syndrome, non-thyroidal illness syndrome). Reverse T3 is generally metabolically inactive, although weak nuclear binding activity has been reported. Secretion and plasma levels of TSH are inversely related to circulating levels of FT3 and FT4. The inhibitory feedback action of FT3 and FT4 involves a direct action of these hormones on the pituitary gland without involving the hypothalamus. Therefore, secretion of TSH is regulated directly by the ambient intrapituitary T3 concentration and intrapituitary deiodination of T4 to T3 by type II monodeiodinase activity.77 A progressive decline in the T4 secretion rate with increasing age partially reflects maturational changes in thyrotropin-releasing hormone (TRH) and TSH secretion.27,32 A circadian variation of circulating TSH has been found in normal children and adults.77,78 A peak TSH concentration (≈3-4 mU/L) develops between 10:00 pm and 4:00 am and is about twofold higher (50%-300% higher) than the afternoon (2:00-6:00 pm) nadir values. This nocturnal TSH surge is not directly related to sleep; it is blunted or absent in central (secondary or tertiary) hypothyroidism but maintained in primary hypothyroidism. The circadian pattern of TSH is not yet present in neonates but has been noted to be present in infants as young as 4 months of age. In children age 1 year and older, the am to pm TSH ratio can be useful in identifying mild primary hypothyroidism and in differentiating this from central hypothyroidism.78 In most children with idiopathic hypopituitarism, TSH release after TRH administration is normal, indicating impaired TRH secretion rather than a primary TSH deficiency (of note, TRH is not available in the United States).97 Thyrotropin-releasing hormone–mediated TSH release can be augmented by administration of theophylline or estradiol and may be blunted by the administration of l-dopa, somatostatin, or glucocorticoids. However, clinically significant hypothyroidism rarely occurs after prolonged administration of glucocorticoids or adrenocorticotropic hormone (ACTH). With the development of sensitive methods, more tests to determine thyroid function and disease have become available in hospitals and reference laboratories (Box 97-2). Concomitantly, many previously used thyroid function tests are now obsolete, such as the protein-bound iodine test. Total plasma or serum TSH, T4, T3, and rT3 can be determined by specific competitive assays. These procedures require a very small quantity of blood (<50 µL), an advantage exploited for screening neonates for hypothyroidism. Determination of TSH is the most sensitive test to detect primary hypothyroidism, or thyroid gland failure.8 The plasma pool of T4 constitutes a large protein-bound reservoir; this pool turns over slowly. Therefore, T4 measurement usually reflects the adequacy of the hormonal supply. The normal level of T4 is age dependent in infancy and childhood, particularly during the neonatal period. T4 reaches a peak concentration shortly after birth and declines slowly, gradually approaching the adult normal range in puberty.27 The range of normal levels for each age group is also wide (Figure 97-2). However, it should be kept in mind that more than 99% of circulating T4 is bound to serum thyroid hormone–binding proteins. Any change or abnormality in the concentration of these proteins, particularly TBG, can affect the T4 level. Several clinical situations and pharmacologic agents can alter the levels of TBG or TTR. Amiodarone, an antiarrhythmic drug, contains covalently bound iodine and can alter thyroid test results.10 It inhibits the hepatic conversion of T4 to T3 and decreases the clearance of T4. FT4 and rT3 are increased, and T3 and FT3 are decreased. Because of its high content of iodine, amiodarone can also result in either hypothyroidism or hyperthyroidism and may cause congenital goitrous hypothyroidism when it is administered to pregnant women. This effect is analogous to direct exposure of the fetus or infant to high doses of iodine. When TSH becomes elevated, the percentage of T3 produced by the thyroid gland is increased. Serum concentration of T3 can vary from day to day. Because of the overall small quantity of T3 in serum, its level may not fall below the normal range until T4 is critically low. Therefore, serum T3 is not very useful in evaluation of patients for possible hypothyroidism. T3 is physiologically low in the fetus and cord blood, but rises promptly after birth to levels greater than those in older children and adults. T3 and FT3 are also low during nonthyroidal illness. Normal serum T3 concentrations vary among laboratories, but generally range from about 100 to 220 ng/dL in children and 80 to 200 ng/dL in adults.8 Most hormonally inactive rT3 is derived from deiodination of T4 and represents a deiodination rate similar to that of T4-to-T3 conversion in normal adults. Serum half-life is very short, less than one half that of T3. Concentration of rT3 usually parallels that of T3. However, rT3 is disproportionately high in the fetus, in the early neonatal period, and in severe nonthyroidal illness, probably reflecting altered tissue metabolism of T4.8 Normal adult serum level of rT3 is 30 to 80 ng/dL. FT4 in serum can be estimated by direct dialysis. This method depends on the FT4 concentration being governed by equilibrium in levels of binding proteins, protein-bound T4, and FT4, following the law of mass action. In clinical settings, FT4 does not depend on the T4-binding capacity as such because a change in such a capacity is soon compensated for by a change in the amount of T4 released from the thyroid. To estimate FT4, serum is placed in a dialysis cell on one side of a semipermeable dialysis membrane, with a buffer solution on the other side. T4 equilibrates across the membrane. Bound T4 remains with serum, and free T4 crosses the membrane and enters the buffer solution. T4 is measured in the buffer solution, and the amount corresponds to the level of endogenous FT4. FT3 levels in the serum can be measured in the same dialysate as that used for FT4. FT4 by dialysis may be artifactually elevated in familial dysalbuminemic hyperthyroxinemia.39 The principle of analogue methods is that the rate of binding of labeled hormones to antibodies depends on the FT4 concentration during a timed incubation. Analogue methods are less expensive, can be performed more rapidly than the direct dialysis method, and are acceptable for routine testing in children. However, FT4 analogue methods may not provide an accurate assessment of FT4 in some neonates, patients receiving medications that interfere with T4 binding, chronically or critically ill neonates or children, or patients with very low levels of TBG.94 When hypothalamic or pituitary hypothyroidism is suspected, the definitive test to measure the FT4 is by direct dialysis. This test is readily available in specialized commercial laboratories. Because there is a delay in obtaining results, the specimen should be collected and T4 therapy started. If there is an associated ACTH or cortisol deficiency, hydrocortisone therapy should be started at least 6 to 8 hours before T4 therapy is initiated.45 Measurements of TSH and FT4 are the most important tools for screening thyroid function. Serum TSH is measured by specific competitive-binding isotopic and nonisotopic methods, and values are expressed in milliunits per liter (mU/L) of an international reference standard. TSH is the most sensitive test for primary hypothyroidism at any age and is used in many neonatal thyroid screening programs. Serum TSH is also an indicator of the adequacy of thyroid hormone replacement therapy and is used in assessment of the hypothalamic-pituitary-thyroid axis by the TSH surge test and the TRH test (see Thyrotropin-Releasing Hormone). The normal adult value is 0.2 to 3.0 mU/L.8 TSH is elevated in primary hypothyroidism. A surge of TSH release also occurs at parturition and reaches a peak within 2 hours after birth. TSH in the cord serum and the infant’s serum after the first day of postnatal life is usually less than 20 mU/L. Therefore, TSH is the most important test to screen for primary congenital hypothyroidism. The target range for TSH during thyroid hormone therapy for primary hypothyroidism is 0.5 to 2 mU/L. The highly sensitive TSH assay can also be used in identifying individuals with thyrotoxicosis in whom TSH is suppressed to less than 0.01 mU/L. The development of sensitive and specific assays to quantitate thyroid hormone and TSH has largely obviated the need for radioiodine uptake studies in childhood. As a result, radioactive thyroid scans are rarely performed in infants and children. Measurement of TG concentration can identify the presence or absence of thyroid glandular tissue. Ultrasonography of the thyroid gland is useful for visualization of normal-sized glands and goiters. It is also used to distinguish solid and cystic nodules of the thyroid. Ultrasonography can identify dysgenetic thyroid glands.68 Slower correction of TSH may be seen with dysgenetic glands than with eutopic glands. Ultrasonography should be used as the first imaging tool, with a scan performed to distinguish agenesis from ectopia. However, hypoplastic or ectopic glands may be missed by this technique. When a scan is necessary, 123I or 99mTc should be used to reduce radiation exposure to the child. The half-life of 123I is 13.3 hours, compared with 8 days for 131I. A bolus infusion of TRH (7 µg/kg of body weight) results in increased TSH that peaks at 20 to 30 minutes. A peak increment of 10 to 30 mU/L is seen in healthy children, with a decline to basal TSH levels by 2 to 3 hours. Serum T3 levels peak at 3 to 4 hours and T4 levels at 6 to 9 hours after TRH. Therefore, the TRH test can be used to examine responsiveness of both TSH to TRH and of thyroid gland to TSH when necessary. Patients with primary hypothyroidism have an exaggerated TSH response. Patients with hyperthyroidism and those receiving T4 replacement do not respond to TRH stimulation because of chronic suppression of TSH by thyroid hormone.45 The TRH test may be helpful in confirming central hypothyroidism (pituitary or hypothalamic) in some patients. Those with secondary (pituitary) hypothyroidism do not respond to TRH.45 In some patients with tertiary hypothyroidism (hypothalamic TRH deficiency), a delayed response of TSH to TRH stimulation is seen, so TSH at 60 minutes is higher than at 20 to 30 minutes. However, many patients with tertiary hypothyroidism have a normal response to TRH. Central hypothyroidism is diagnosed in infants who have low or low-normal FT4, no TSH elevation, and other common features of hypopituitarism (e.g., hypoglycemia, microphallus). More subtle central hypothyroidism (FT4 in the lowest third of the normal range) can be confirmed in children older than 1 year by the am to pm TSH ratio or the TSH surge test.77,78 This test is not useful in infants before development of the circadian pattern of TSH secretion. The TSH surge test is performed by obtaining blood for TSH assay at the usual time of the nadir of the circadian variation of TSH (the mean of two or three samples between 10:00 am and 6:00 pm) and again at the usual time of peak TSH secretion (the mean of the three highest sequential samples between 10:00 pm and 4:00 am). The mean nadir and peak TSH values are calculated. Normal night-time (peak) TSH values are 50% to 300% higher than those (nadir) obtained during the day. Blunting or absence of this rise confirms central hypothyroidism.77 At age older than 1 year, with FT4 less than 1.2 mg/dL, an 8 am to 4 pm TSH ratio less than 1.3 also confirms central hypothyroidism.78 Factors such as FOXE1, NKX2-1, PAX8, TBX1, HOXA3, FGF10, and HHEX are involved in stem cell differentiation into thyroid follicular cells (Table 97-1).5,7,30,44 Activin A, insulin, and IGF-I are among the hormones required for generation of thyroid cells.23 The major portion of the human thyroid originates from the median anlage, the tissue that arises from the pharyngeal floor (toward the back of the future tongue) and is identifiable in the 17-day-old embryo.29 The median anlage is initially in close contact with the endothelial tubes of the embryonic heart. With the descent of the heart, the rapidly growing median thyroid is progressively pulled caudally until it reaches its final position in front of the second to sixth tracheal ring by 45 to 50 days of gestation. Abnormal descent leads to ectopic location of the thyroid gland.63 The pharyngeal region contracts to become a narrow stalk called the thyroglossal duct, which subsequently atrophies. The descent of the heart may influence the downward movement of the thyroid because of topographic contact. The median anlage usually grows caudally so that no lumen is left in the tract of its descent. An ectopic thyroid gland or persistent thyroglossal duct or cyst results from abnormalities of thyroid descent. Lateral parts of the descending median anlage expand to form the thyroid lobes and the isthmus.29 TABLE 97-1 Inherited Disorders of Thyroid Metabolism Adapted from Knobel M, Medeiros-Neto G. An outline of inherited disorders of the thyroid hormone generating system. Thyroid. 2003;13:771. By the latter part of the 10th week of gestation, the histogenesis of the thyroid is virtually complete, although the follicles do not contain colloid.29 A single layer of endothelial cells surrounds the follicular lumen. T4 has been detected in the serum of a 78-day-old fetus. At this age, the fetal thyroid is capable of trapping and oxidizing iodide.45 Therefore, the fetal thyroid begins to secrete thyroid hormone and contributes to the fetal circulation of thyroid hormone by the beginning of the second trimester. At the same time as the development of the thyroid gland, the fetal pituitary and hypothalamus are also forming and beginning to function. The anterior pituitary gland is derived from the Rathke pouch, which originates at the roof of the pharynx. Histologic differentiation of pituitary cells can be observed by 7 to 10 weeks of gestation, and TSH can be detected in fetal blood by 10 to 12 weeks.50 The hypothalamus develops from the ventral portion of the diencephalon. Thyrotropin-releasing hormone has been found in fetal whole-brain extracts by 30 days and in the hypothalamus by 9 weeks of gestation. Knowledge regarding the genetic basis of normal thyroid physiology and disease has been rapidly developing and will continue to advance (see Table 97-1).48 For additional updates, the reader can consult the following websites: www.ncbi.nlm.nih.gov/PubMed (accessed November 8, 2013), www.ncbi.nlm.nih.gov/Omim (accessed November 8, 2013), and http://medicine.cf.ac.uk/ (accessed November 8. 2013).
Thyroid Disorders in the Neonate
Physiologic Action of Thyroid Hormones
Functions of the Thyroid Gland
Neurologic Effects
Cellular Metabolism
Protein and Lipid Metabolism
Growth and Development
Synthesis, Release, Transport, and Use of Thyroid Hormones
Iodine Metabolism
Secretion of Triiodothyronine and Thyroxine
Monodeiodination of Thyroxine
Regulation of Thyroid Function
Laboratory Tests Used in the Diagnosis of Thyroid Disease in Infancy and Childhood
Concentration of Circulating Thyroid Hormones
Thyroxine
Drug Effects on Thyroid Concentrations
Triiodothyronine
Reverse Triiodothyronine
Free Hormones
Thyrotropin
Thyroid Imaging
Thyrotropin-Releasing Hormone
AM to PM Thyroid-Stimulating Hormone Ratio, Thyroid-Stimulating Hormone Surge Test
Embryogenesis
Hypothalamic-Pituitary Development
Gene
Inheritance
Combined pituitary hormone deficiency
Mutations of LHX3, LHX4, HESX1, PROP1, POU1F1
AR or AD
Isolated TSH deficiency
TRH, TSH beta-subunit, TRHR mutations
AR
TSHR
Loss-of-function TSHR mutation
Resistance to TSH with normal TSHR
Gain-of-function TSHR mutation
GNAS1 mutations
AR or AD
AD
AD
Thyroid Gland Development
Mutations of NKX2 (prior TTF1), FOXE1 (prior TTF2), PAX8, TBX1, HOXA2, HHEX, BCL2, SHH
AR or AD
Organification
Iodide transport, Pendred syndrome
PDS or Iodide symporter NIS SLC26A4
AR
TPO
TPO mutations
THOX2
AR
Thyroid NADPH oxidase
DUOX1 and DUOX2 mutations
TG
TG mutations
AR or AD
Iodide cycling
Defect not known
?AR
Deiodinase
DEHAL1
AR
Thyroid Hormone Transport
TBG
TBG deficiency, partial or complete X-linked recessive
TBG excess
X-linked recessive
X-linked recessive
TTR
TTR mutations
AD
Albumin
Albumin mutations
AD
Thyroid Hormone Action
THR-beta
MCT8
SECISBP2
AD
X-linked
AR
Generalized Thyroid Hormone Resistance
THR mutations
THR-beta
AD
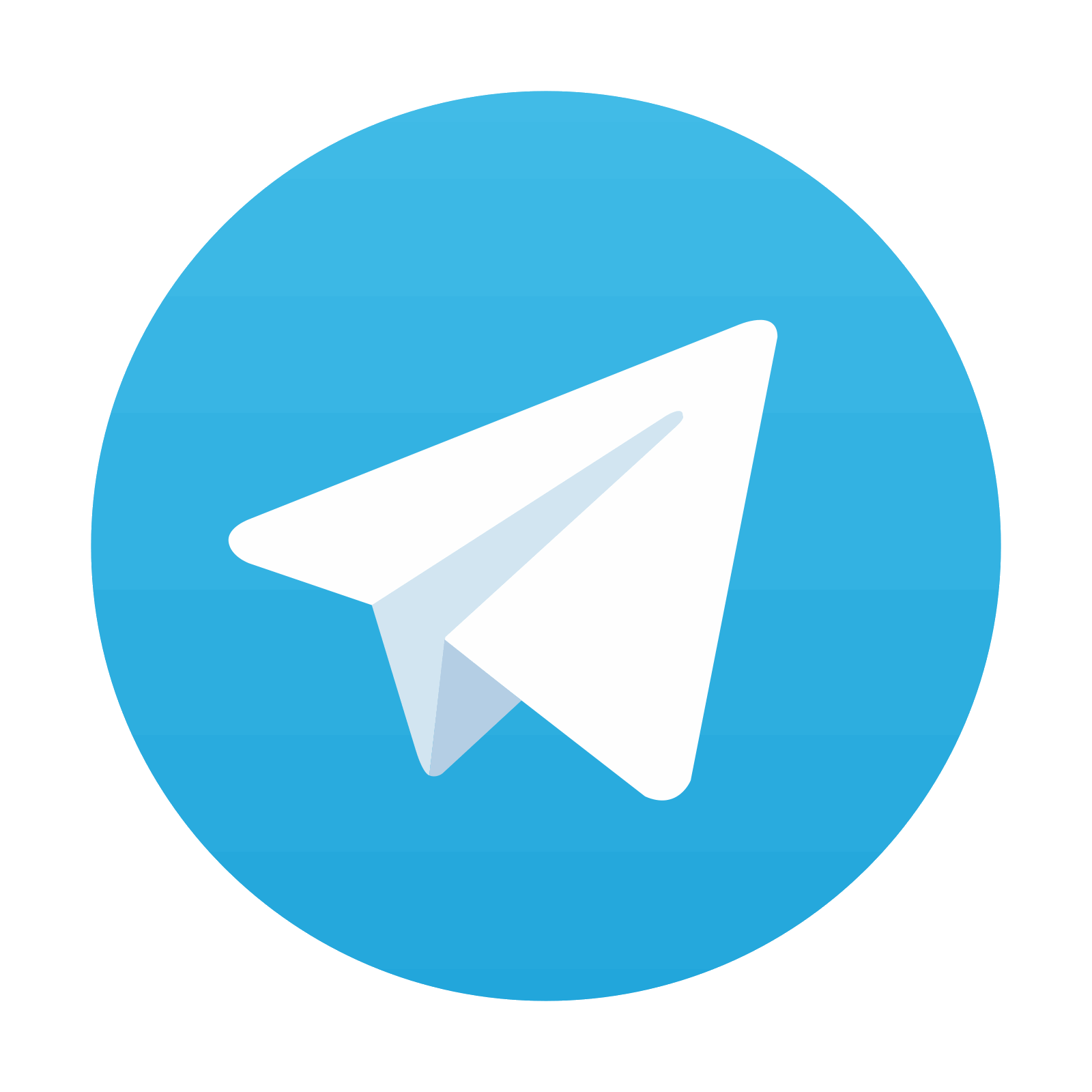
Stay updated, free articles. Join our Telegram channel
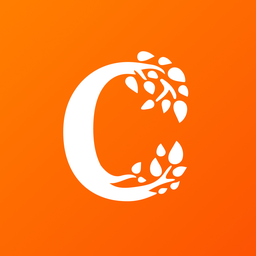
Full access? Get Clinical Tree
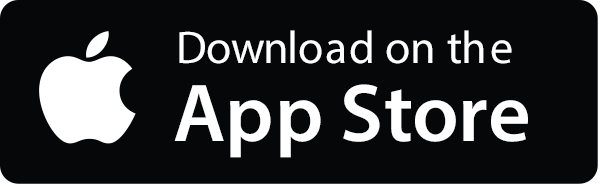
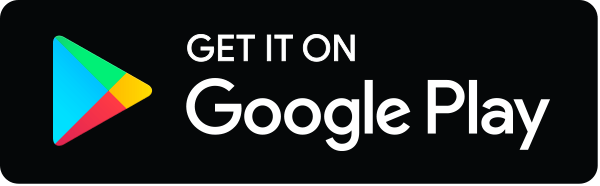