Respiratory Distress Syndrome in the Neonate
Enormous strides have been made in understanding the pathophysiology of respiratory distress syndrome (RDS) and the role of surfactant in its cause and treatment. Nevertheless, RDS, formerly referred to as hyaline membrane disease, remains a dominant clinical problem encountered among preterm infants.9 The greatly improved outcome in RDS can be attributed primarily to the introduction of pharmacologic acceleration of pulmonary maturity and the development of surfactant replacement therapy.31,58
Incidence
Respiratory distress syndrome is one of the most common causes of morbidity in preterm neonates, although lack of a precise definition in infants with very low birth weight necessitates cautious interpretation of statistics regarding incidence, mortality, and outcome.11,59 The diagnosis can be established pathologically or by biochemical documentation of surfactant deficiency; nonetheless, most series refer only to a combination of clinical and radiographic features. Without biochemical evidence of surfactant deficiency, it is difficult to clinically diagnose RDS in infants with extremely low birth weight. The term respiratory insufficiency of prematurity had been widely used in some centers for infants who need oxygen and ventilator support in the absence of typical radiographic evidence of RDS. Recently, the term respiratory instability of prematurity has been proposed to describe very low birth weight infants who require some respiratory support, but may have additional contributing factors such as inconsistent central respiratory drive or poor inspiratory effort.11
Respiratory distress syndrome occurs throughout the world and has a slight male predominance.5 The greatest risk factors appear to be young gestational age and low birth weight; however, European descent, late preterm delivery (35 to 36 weeks), or elective delivery in the absence of labor are also prominent risk factors.5,48 Given the associated adverse respiratory outcomes of late preterm and early term births,68,103 initiatives to delay elective deliveries until 39 weeks’ gestation have been successful in reducing pulmonary and nonpulmonary morbidity in these infants.28,74,94 Other risk factors include maternal diabetes and perinatal hypoxia-ischemia.
The incidence of RDS is inversely proportional to gestational age: 95% to 98% of infants born at 22 to 24 weeks’ gestation have RDS, decreasing to approximately 25% in infants with birth weights between 1251 and 1500 g.32,92 Even infants of 34 weeks’ gestation and above, especially males of European descent, have a discernible risk of RDS that decreases from 5% at 34 weeks to less than 1% at 37 weeks (Figure 72-1).5

Pathophysiology
The lungs of infants who succumb from RDS have a characteristic uniformly ruddy and airless appearance, macroscopically resembling hepatic tissue. On microscopic examination, the striking feature is diffuse atelectasis such that only a few widely dilated alveoli are readily distinguishable (Figure 72-2). An eosinophilic membrane lines the visible airspaces that usually constitute terminal bronchioles and alveolar ducts. This characteristic membrane (from which the term hyaline membrane disease is derived) consists of a fibrinous matrix of materials derived from the blood and contains cellular debris derived from injured epithelium. The recovery phase is characterized by regeneration of alveolar cells, including the type II cells, with a resultant increase in surfactant activity. The development of RDS begins with impaired or delayed surfactant synthesis and secretion followed by a series of events that may progressively increase the severity of the disease for several days (Figure 72-3). Surfactant synthesis is a dynamic process that depends on factors such as pH, temperature, and perfusion and may be compromised by cold stress, hypovolemia, hypoxemia, and acidosis. Other unfavorable factors, such as exposure to high inspired oxygen concentration and the effects of barotrauma and volutrauma from assisted ventilation, can trigger the release of proinflammatory cytokines and chemokines and further damage the alveolar epithelial lining, resulting in reduced surfactant synthesis and function. The leakage of proteins such as fibrin in the intra-alveolar space further aggravates surfactant deficiency by promoting surfactant inactivation. Deficiency of surfactant and the accompanying decrease in lung compliance lead to alveolar hypoventilation and ventilation-perfusion () imbalance.


Severe hypoxemia and systemic hypoperfusion result in decreased oxygen delivery with subsequent lactic acidosis secondary to anaerobic metabolism. Hypoxemia and acidosis also result in pulmonary hypoperfusion secondary to pulmonary vasoconstriction, and the result is a further aggravation of hypoxemia due to right-to-left shunting at the level of the ductus arteriosus, the foramen ovale, and within the lung itself.
The relative roles of surfactant deficiency and pulmonary hypoperfusion in the overall clinical picture of RDS vary somewhat with each patient. The natural history of RDS is now almost invariably altered by a combination of antenatal corticosteroid administration, exogenous surfactant therapy, and assisted ventilation.
Heritability of Respiratory Distress Syndrome
A genetic contribution to the risk of RDS has been suggested in twin studies where the concordance of RDS in monozygotic twins is greater than that of dizygotic twins and with isolated reports of recurrence in families.53,61,70 With the application of molecular techniques, the contribution of genetic variations (polymorphisms, mutations) to the pathogenesis of respiratory disorders in newborns is rapidly emerging.22,99
Mutations in genes encoding surfactant protein-B (SP-B), surfactant protein-C (SP-C), the ATP binding cassette subfamily A, member 3 (ABCA3), and the thyroid transcription factor (NKX2-1) represent rare monogenic causes of RDS.22,43,73,85 Inherited SP-B deficiency is a recessive disorder that presents as severe respiratory failure in the immediate newborn period and is unresponsive to standard intensive care interventions. With a disease frequency of approximately 1 per million live births, the absence of SP-B, the presence of an incompletely processed proSP-C, and a generalized disruption of surfactant metabolism cause surfactant dysfunction and the clinical syndrome. Dominant mutations in the SP-C gene are present in significantly less than 0.1% of the population99 and typically result in interstitial lung disease in infants older than 1 month of age, although an “RDS-like” presentation has been described.18 The accumulation of misfolded proSP-C within cellular secretory pathways results in activation of cell stress responses and apoptosis and impaired surfactant function.69 Recessive, deleterious mutations in ABCA3 are present in approximately 4% of the population99 and have been identified in association with lethal RDS in newborns and with chronic respiratory insufficiency in children.26,90 Although the frequency of disease is unknown, accumulating experience suggests that ABCA3 deficiency may be the most common of these disorders of surfactant homeostasis. Data from humans and mice suggest that ABCA3 mediates surfactant phospholipid transport (primarily phosphatidylcholine and phosphatidylglycerol) into lamellar bodies, and thus dysfunction of phospholipid transport into the lamellar body leads to reduced surfactant function.33,38,65,100 Dominant mutations in NKX2-1 were first identified in the context of benign hereditary chorea, but since then, have also been recognized in lethal and nonlethal neonatal RDS as well as interstitial lung disease in older children.25,43,102 A triad of neurologic disease, characterized by hypotonia, developmental delay and movement disorders, congenital hypothyroidism, and RDS has been termed the brain-thyroid-lung syndrome, but any single organ presentation or combination thereof may be the initial and only manifestation of the syndrome.43 Mutations in NKX2-1 are rare, but the frequency of disease is unknown. Disruption in structural lung development and/or decreased expression of surfactant-associated genes are the postulated mechanisms of disease.
Large-scale cohort-based resequencing efforts have demonstrated an over-representation of single, heterozygous mutations in ABCA3 in newborns 34 weeks and greater with RDS, suggesting that these mutations are modifiers for the risk or severity of respiratory disease in developmentally susceptible newborns.39,99 These efforts have failed to identify an unequivocal contribution of rare or common variants in the surfactant protein-B or -C genes to RDS in newborns, suggesting that if these genes play a role in RDS, it is likely to be through interactions with variants in other lung-associated genes.44 Exome and genome sequencing are becoming more accessible and will yield additional insights into candidate genes that account for the heritability of RDS.30,55
Prevention: Pharmacologic Acceleration of Pulmonary Maturation
In the early 1970s, Liggins, while studying the effects of steroids on premature labor in lambs, noticed the lack of RDS and increased survival in preterm animals prenatally exposed to steroids. The effects of various catecholamines as well as aminophylline and thyroid hormone have been studied; however, the most successful method to induce fetal lung maturation is prenatal corticosteroid administration (see Chapter 70).63
If premature delivery of any infant appears probable or necessary, lung maturity can be hastened pharmacologically. Accelerated lung maturation occurs with physiologic stress levels of corticosteroids, via receptor-mediated induction of specific developmentally regulated proteins, including those associated with surfactant synthesis. Steroids, when administered to the mother at least 24 to 48 hours before delivery, decrease the incidence and severity of RDS. Corticosteroids appear to be most effective before 34 weeks of gestation and when administered at least 24 hours and no longer than 7 days before delivery. Because corticosteroid therapy for less than 24 hours is still associated with significant reductions in neonatal mortality, RDS, and intraventricular hemorrhage (IVH), antenatal steroids should always be considered unless immediate delivery is anticipated.80 The use of antenatal corticosteroids before 24 weeks and after 34 weeks’ gestation remains controversial.
Less clear are the effects of repeated courses of antenatal corticosteroids on the short- and long-term outcomes of preterm infants. Decreased fetal growth and poorer neurodevelopmental outcomes have been reported in retrospective clinical studies.12 Therefore, until prospective data are available, caution should be exercised with the widespread use of multicourse therapy.
Concern about the possibility of increased infection in mother or infant appears to be unfounded. Indeed, even when corticosteroids are administered to women with prolonged rupture of membranes, there is no evidence of increased risk of infection, and the neuroprotective effects of corticosteroids are still evident. Maternal steroids may induce an increase in total leukocyte and immature neutrophil counts in the infant, which should be considered if neonatal sepsis is suspected.13
There is proven benefit from the combined use of prenatal corticosteroids and postnatal surfactant therapy in preterm infants (see Surfactant Therapy). Their effects appear to be additive in improving lung function. Antenatal steroids induce structural maturation of the lung, as evidenced by physiologic and morphometric techniques, that is not secondary to increases in alveolar surfactant pool sizes.50 These structural changes translate into improved physiologic properties of the lung, such as increased lung volume, increased lung compliance, and increased response to exogenous surfactant treatment.
Antenatal corticosteroids appear to reduce the incidence of other co-morbidities associated with prematurity including intracranial hemorrhage and necrotizing enterocolitis.62,80 The beneficial effect on intracranial hemorrhage does not correlate directly with improved pulmonary morbidity and may be secondary to stabilization of cerebral blood flow or a steroid-induced maturation of vascular integrity in the germinal matrix, or both.
In the 1990s, the observation that thyroid hormone could augment lung development and surfactant production in vitro prompted trials of antenatally administered thyrotropin releasing hormone (TRH). However, these trials failed to demonstrate a benefit and also raised concerns for adverse consequences on neurodevelopment, thereby significantly dampening enthusiasm for this therapy.23
Clinical Features
The classic clinical presentation of RDS includes grunting respirations, retractions, nasal flaring, cyanosis, and increased oxygen requirement, together with diagnostic radiographic findings and onset of symptoms shortly after birth. The respiratory rate is usually regular and increased well above the normal range of 30 to 60 breaths per minute. These infants usually show progression of respiratory symptoms and require supplemental oxygen. The presence of apneic episodes at this early stage is an ominous sign that could reflect thermal instability or sepsis, but more often is a sign of hypoxemia and respiratory failure. This characteristic picture is modified in many infants with low birth weight as a result of the early administration of exogenous surfactant and immediate assisted ventilation.
Retractions are prominent and are the result of the compliant rib cage collapse on inspiration as the infant generates high negative intrathoracic pressures to expand the poorly compliant lungs. The typical expiratory grunt is an early feature and may subsequently disappear. Grunting is thought to result from partial closure of the glottis during expiration and in this way acts to trap alveolar air and maintain functional residual capacity (FRC). Although these signs are characteristic for neonatal RDS, they can result from a wide variety of nonpulmonary causes, such as hypothermia, hypoglycemia, anemia, polycythemia, or sepsis; furthermore, such nonpulmonary conditions can complicate the clinical course of RDS.
Cyanosis is a consequence of right-to-left shunting in RDS and is typically relieved by administering a higher concentration of oxygen and ventilatory support. Impaired cardiac output resulting from respiratory effort that is asynchronous with the ventilator may further impede oxygen delivery and lead to poor peripheral perfusion or cyanosis. The consistency of the arterial waveform with invasive blood pressure monitoring or the pulse signal with oxygen saturation monitoring can provide information about the effectiveness of cardiac output. Acrocyanosis of the hands and feet is a common finding in normal infants and should not be confused with central cyanosis, which always must be investigated and treated. Peripheral edema, often present in RDS, is of no particular prognostic significance unless it is associated with hydrops fetalis.
During auscultation of the chest, breath sounds are widely transmitted and cannot be relied upon to reflect pathologic conditions. Non-homogeneous aeration plus elevated endogenously or exogenously generated intrathoracic pressures can cause pulmonary air leaks. Thus unilaterally decreased breath sounds (with mediastinal shift to the opposite side) or bilaterally decreased air entry could indicate pneumothorax, and immediate transillumination must be performed. Chest radiography is also needed to confirm endotracheal tube placement and if air entry sounds are asymmetric. The murmur of a PDA is most often audible during the recovery phase of RDS, when pulmonary vascular resistance has fallen below systemic levels and there is left-to-right shunting. Distant, muffled heart sounds should alert one to the possibility of pneumopericardium. Percussion of the chest is of no diagnostic value in preterm infants.
A constant feature of RDS is the early onset of clinical signs of the disease. Most infants present with signs and symptoms either in the delivery room or within the first 6 hours after birth. Inadequate observation can lead to the impression of a symptom-free period of several hours. The uncomplicated clinical course is characterized by a progressive worsening of symptoms, with a peak severity by days 2 to 3 and onset of recovery by 72 hours. Surfactant therapy often greatly shortens this course. When the disease process is severe enough to require assisted ventilation or is complicated by the development of air leak, significant shunting through a PDA, or early signs of BPD, recovery can be delayed for days, weeks, or months (see Figure 72-3). In the most affected patients, the transition from the recovery phase of RDS to BPD is clinically imperceptible.
Radiographic Findings
The diagnosis of RDS is based on a combination of the previously described clinical features, evidence of prematurity, exclusion of other causes of respiratory distress, and characteristic radiographic appearance (see Chapter 40). The typical radiographic features consist of a diffuse reticulogranular pattern, giving the classic ground-glass appearance, in both lung fields with superimposed air bronchograms (Figure 72-4). Although the radiologic appearance of RDS is typically symmetric and homogeneous, asymmetry has also been described, especially if surfactant therapy has been administered preferentially to one side.

The reticulogranular pattern is primarily caused by alveolar atelectasis, although there may be some component of pulmonary edema. The prominent air bronchograms represent aerated bronchioles superimposed on a background of nonaerated alveoli. An area of localized air bronchograms may be normal in the left lower lobe overlying the cardiac silhouette, but in RDS they are widely distributed, particularly in the upper lobes. In the most severe cases, a complete opacification of the lungs can be observed, with total loss of the cardiac borders. Heart size is typically normal or slightly increased. Cardiomegaly may herald the development of congestive cardiac failure from a PDA. After the administration of exogenous surfactant therapy, the chest radiograph usually shows improved aeration of the lungs bilaterally; however, asymmetric clearing of the lungs may occur.
The radiographic appearance of RDS, typical or atypical, cannot be reliably differentiated from that of neonatal pneumonia, which is most commonly caused by group B streptococcal or gram-negative (e.g., E. coli) infection. This problem has been the major reason for the widespread use of empiric antibiotics in the initial management of infants with RDS. Infants with RDS reportedly have a larger thymic silhouette than infants of comparable size without RDS. This supports the theory that patients with RDS have had reduced exposure to endogenous corticosteroids during fetal life.
Echocardiographic evaluation of selected infants with RDS may be of value in the diagnosis of a PDA, to quantitate elevations in pulmonary artery pressure, to assess cardiac function, and to exclude congenital heart disease (e.g., obstructed total anomalous pulmonary venous return) (see Chapter 83).
Treatment
Therapy for RDS includes careful application of general supportive measures supplemented by surfactant therapy and assisted ventilation. Close and detailed supervision of small infants requires a dedicated, trained staff experienced and interested in problems specific to the newborn and skillful in the unique technical procedures involved, such as CPAP administration, endotracheal intubation, titration of invasive and noninvasive respiratory support, and assessment of neonatal blood gases.
Positive-Pressure Ventilation
Delivery of distending pressure to maintain airway and alveolar expansion through invasive and noninvasive support is the mainstay of treatment for RDS (see Chapter 73). The most common approaches of invasive mechanical ventilation via an endotracheal tube utilize a time-cycled, pressure-limited mode, or volume-controlled mode with synchronized ventilated breaths. Alternatively, high-frequency jet ventilation or high-frequency oscillatory ventilation is utilized both as a primary means of ventilation and as rescue when conventional ventilation has failed.
There has been much enthusiasm for noninvasive methods of ventilation, especially nasal continuous positive airway pressure (nCPAP). The benefits of CPAP include maintenance of a constant airway opening pressure, establishment and maintenance of functional residual capacity, reduction of pharyngeal or laryngeal obstruction, improvement of oxygenation, and release of surfactant stores.1,40,93 When compared to invasive mechanical ventilation, nCPAP reduced barotrauma, volutrauma, airway damage, and risk of secondary infections, as well as enhanced mucociliary transport.20,41
Nasal CPAP used in combination with surfactant for the treatment of RDS among premature infants is well established. Early studies demonstrated reduced need for mechanical ventilation among very preterm infants treated with surfactant and nCPAP.98 Recently, several randomized clinical trials have addressed the early-life respiratory management of extremely premature infants (25 to 28 weeks) at risk for RDS. Taken together, these trials demonstrate that a subset of extremely premature infants, especially those exposed to antenatal corticosteroids, can be stabilized with nCPAP shortly after birth and may avoid intubation, ventilation, and surfactant altogether.24,29,67,71,82 However, methods to identify this subset of infants before delivery or soon after birth are not well characterized.35
Although some extremely premature infants can be treated with nCPAP, many infants fail this strategy because of persistent and severe apnea, desaturation, bradycardia, or respiratory acidosis. Noninvasive positive-pressure ventilation (NIPPV) combined nCPAP with intermittent ventilator breaths and can be administered through short binasal prongs. Noninvasive positive-pressure ventilation increases tidal and minute volumes,66 improves lung recruitment, decreases work of breathing,2 and may reduce apnea of prematurity.64,78 Synchronized NIPPV is effective in weaning premature infants with RDS from mechanical ventilation14,34,52,57,66 and may improve pulmonary outcomes.15,79
Surfactant Therapy
First introduced in 1990, no intervention in the past 20 years has had more impact on the care of newborns with RDS than surfactant replacement therapy. A thorough history of the development of surfactant replacement therapy can be found in Chapter 70 and reference 42. The discovery that surfactant deficiency was key in the pathophysiology of RDS led several investigators to administer artificial aerosolized phospholipids to infants with RDS. In these early studies, only limited therapeutic success was encountered. In contrast, animal models in which natural surfactant compounds were used yielded more promising results.
Because intact lung preparations were not available in Japan, Fujiwara and co-workers developed a mixture of both natural and synthetic surface-active lipids for use in humans.36 Such a mixture might also afford alveolar stability with less potential risk for a reaction to foreign protein than would be the case with exclusively natural surfactant. When administered to an initial group of 10 preterm infants with severe RDS who were not improving despite artificial ventilation, a single 10-mL dose of surfactant instilled into the endotracheal tube resulted in a dramatic decrease in inspired oxygen and ventilator pressures. None of the infants in this uncontrolled series subsequently succumbed from RDS. Recovery, however, was complicated by clinical evidence of a PDA in nine of the infants, possibly the result of a prompt fall in pulmonary vascular resistance with the resultant left-to-right shunting after surfactant therapy.
Endotracheal administration of exogenous surfactant decreases complications of respiratory distress syndrome among premature infants.49 Multiple studies have employed various combinations of prevention (delivery room administration), early treatment (within first few hours of life), and rescue (administration for established RDS) protocols using synthetic and mixed natural-synthetic preparations.49,88,89 Synthetic-only preparations used protein-free synthetic phospholipid products that contain alcohol to act as a spreading agent for dipalmitoyl phosphatidylcholine at the air-fluid-alveolar interface.75 The mixed-surfactant preparations contain extracts of calf lung lavage or minced bovine or porcine lung. Direct comparison between synthetic and natural preparations has revealed a more rapid physiologic response after natural (protein-containing) preparations as manifested by the ability to lower inspired oxygen and ventilator pressures.88 Comparison of natural surfactants has shown modest differences in oxygenation during the first few hours after treatment, without a significant effect on morbidity or mortality. Despite differences in their chemical composition and manufacturing methods, currently approved formulations demonstrate comparable clinical efficacy.
All regimens of surfactant therapy appear to decrease the incidence of air leaks and improve oxygenation of ventilated preterm infants (Box 72-1). More strikingly, mortality from RDS, and even overall mortality of ventilated preterm infants, is significantly reduced.87 Early selective surfactant administration (within the first few hours after birth) given to infants with RDS requiring assisted ventilation leads to decreased risk of pneumothorax, pulmonary interstitial emphysema, chronic lung disease, and mortality compared with delaying treatment until RDS is well established.10 Given the increased use of antenatal corticosteroids and success of postdelivery stabilization on nCPAP, selective administration of surfactant to infants requiring intubation is now a preferred alternative to prophylactic surfactant administration.81 In contrast with the impressive improvement in mortality, the incidence of BPD appears unaltered in most studies.49 Although individual studies have shown a decreased incidence of BPD, these results have not been substantiated by meta-analysis of large randomized trials. This could be a consequence of the enhanced survival caused by surfactant administration to infants who are very preterm. Furthermore, many infants who are preterm develop BPD without antecedent RDS.
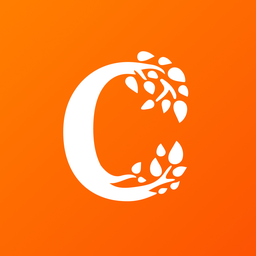
Full access? Get Clinical Tree
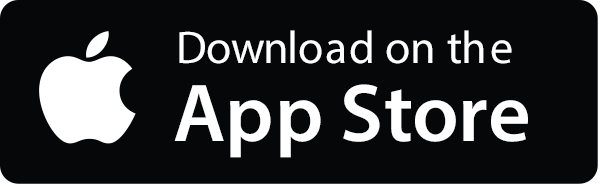
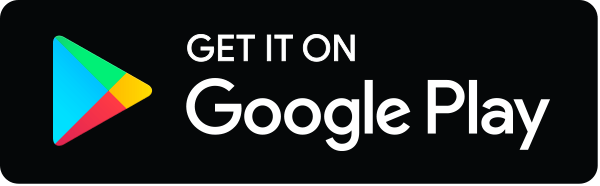