At the time of birth, approximately 20% of very low birth weight (VLBW) infants are small for gestational age (defined as weight less than the 10th percentile).32 After a stay in the neonatal intensive care unit, however, the vast majority of these infants will have experienced poor growth and weigh less than the 10th percentile at 36 weeks’ postmenstrual age. The Eunice Kennedy Shriver National Institute of Child Health and Human Development (NICHD) Neonatal Research Network has published growth outcomes of VLBW infants over the past several decades. Among VLBW infants enrolled in the Neonatal Research Network generic database of morbidity and mortality, the incidence of growth failure at 36 weeks’ postmenstrual age was 97% between 1995 and 199648 and 91% between 1997 and 2002.32 In the most recent report between 2003 and 2007, the incidence of postnatal growth failure decreased to 79%,85 possibly reflecting widespread use of early parenteral nutrition at most neonatal intensive care units as well as a decline in the use of postnatal corticosteroids. The association between weight gain in the neonatal intensive care unit and neurodevelopmental outcomes was evaluated in a cohort of extremely low birth weight (ELBW) infants admitted to centers participating in the NICHD Neonatal Research Network between 1994 and 1995.29 Infants were divided into quartiles based on in-hospital growth velocity; infants in the highest quartile gained an average of 21 g/kg per day, and those in the lowest quartile gained 12 g/kg per day. Compared with infants in the highest quartile of in-hospital weight gain, the odds of cerebral palsy, Bayley Scales of Infant Development (BSID) II mental developmental index (MDI) less than 70, and neurodevelopmental impairment were significantly higher in infants in the lowest quartile of in-hospital weight gain. Similar findings were also observed with in-hospital head growth. A recent evaluation using a more contemporary population and the new edition of the Bayley (BSID III) have reported findings consistent with the earlier work by Ehrenkranz. Other investigators have recently described the association between poor linear growth and neurodevelopmental outcomes.68 As evidence continues to accumulate that early nutritional inadequacies have long-term consequences, optimizing provision of both parenteral and enteral nutrition to high-risk neonates is crucial to ensure the best possible outcomes. Among potential causes of postnatal growth failure are significant protein and energy deficits that occur in the early neonatal period that are difficult to recoup in extremely premature infants.31 Early deficiencies in protein are a particularly important contributor to the poor growth outcomes observed in this population.11,60 Consequently, provision of adequate nutrition to avoid these deficits altogether is necessary to optimize growth in premature infants. Normal human fetal development is characterized by rapid rates of growth and accretion of protein. In fact, the greatest rate of relative protein gain throughout life occurs prior to birth. At 26 weeks’ gestation, the human fetus gains approximately 1.8 to 2.2 g of body protein per day (Figure 43-1), with the placenta supplying about 3.5 g/kg per day of amino acids to the developing fetus. The placental supply of amino acids to the fetus is in excess of that needed for accretion of protein. The extra amino acids are oxidized by the fetus and contribute significantly to fetal energy production. In contrast to the high rate of protein gain in utero, protein losses in extremely premature infants are approximately twofold higher than in term infants.24 In the absence of intravenous amino acids, extremely premature infants lose approximately 1.2 g/kg of protein each day, which corresponds to a daily loss of 1% to 2% of total endogenous body protein stores (see Figure 43-1). Protein requirements and recommendations for protein intake in premature infants have been made based on several different approaches (Table 43-1). Ziegler has quantified protein and energy requirements in relation to body weight using the factorial approach (Table 43-2).103 In the factorial approach, nutrient requirements are determined as the sum of the needs for growth plus needs for replacement of losses. The disadvantage of this approach is that it does not account for nutrient requirements for catch-up growth. It is important to note that protein requirements are inversely related to body weight. Based on the factorial method, protein requirements for infants who weigh less than 1200 grams are estimated at 4.0 g/kg per day. Other investigators have utilized empirical approaches to estimate the amount of protein intake required to duplicate fetal growth.43,69 An expert panel convened by the Life Sciences Research Office (LSRO) concluded that the minimum protein intake for premature infants is 3.4 g/kg per day, and that an intake of 4.3 g/kg per day is tolerated without adverse consequences.45 TABLE 43-1 Protein Requirements and Recommended Intakes TABLE 43-2 Protein and Energy Requirements of Premature Infants Determined by the Factorial Approach Although duplication of in utero weight gain can be achieved at protein intakes of 3 to 3.5 g/kg per day, the European Society of Pediatric Gastroenterology and Nutrition (ESPGHAN) has recently recommended an even higher range of protein intake, taking into account the need to make up for accumulated protein deficits observed in nearly all extremely premature infants. Consequently, the recommendation by ESPGHAN for protein intake in infants weighing up to 1000 g is 4.0 to 4.5 g/kg per day and 3.5 to 4.0 g/kg per day for infants weighing 1000 to 1800 g.3 The ESPGHAN committee further commented that protein intake can be reduced toward discharge if the infant’s growth pattern allows for this. Because neural tissue makes up a greater proportion of body weight, newborns have higher rates of glucose oxidation than adults, with glucose being the primary energy substrate for the brain. The rate of glucose production in term newborns is approximately 3 to 5 mg/kg per minute,23 whereas extremely premature infants have an even higher rate of glucose production of approximately 8 to 9 mg/kg per minute.40 Historically, glucose intolerance in infants with extremely low birth weights was attributed to persistent endogenous hepatic glucose production in the face of increased exogenous supply, insufficient insulin production, or tissue insensitivity to insulin. However, ELBW infants are able to suppress endogenous glucose production when given parenteral glucose.40 Energy balance is a delicate equilibrium between energy intake and energy loss plus storage. Positive energy balance is achieved when exogenous metabolizable energy intake is greater than energy expenditure. Growth is then possible, with the excess energy stored as new tissue, usually fat. If exogenous energy intake is less than expenditure, energy balance is negative, and body energy stores must be mobilized to meet ongoing needs. During the acute phase of disease, the primary goal is avoidance of catabolism. This is difficult for infants with very low birth weights, owing to their higher maintenance energy requirements, lower energy stores, and often reduced intake. The components of estimated energy requirements for growing premature infants is shown in Table 43-3. TABLE 43-3 Estimated Energy Requirements for Growing Premature Infants Lactose is the predominant carbohydrate in human milk (6.2-7.2 g/dL) and supplies 40% to 50% of the caloric content. Lactose is hydrolyzed to glucose and galactose in the small intestine by β-galactosidase (lactase). Intestinal lactase activities in premature infants at 34 weeks’ gestational age are approximately 30% of those of term infants.44 Despite low lactase activities in premature infants, lactose is well tolerated by premature infants, and stable isotope data suggest efficient lactose digestion. However, most premature infant formulas include glucose polymers as a significant source of carbohydrate; these glucose polymers are digested by α-glucosidases, which achieve 70% of adult activity between 26 and 34 weeks’ gestation. In addition, salivary and mammary amylases may contribute to glucose polymer digestion. Glucose polymers have the advantage of increased caloric density without a rise in osmolality, and they may also enhance gastric emptying. The American Academy of Pediatrics1 has recommended an average energy intake of 105 to 130 kcal/kg per day for preterm infants. The ESPGHAN Committee on Nutrition recommendation for energy intake for growing, premature infants with adequate protein intake is 110 to 135 kcal/kg per day.3 Infants who are small for gestational age or infants with diseases that increase energy requirements may need higher intakes to achieve the same growth rates. Infants with growth restriction often require an increased caloric intake for growth because of both higher maintenance energy needs and higher energy costs of new tissue synthesis. The LCPUFAs play an important role in the development of the infant’s brain during the last trimester of pregnancy and also during the first months of life.90 The precursor C18 fatty acids for the n-6 and n-3 LCPUFA series are linoleic acid (C18:2n-6) and α-linolenic acid (C18:3n-3). These are further elongated and desaturated to form other fatty acids, of which arachidonic acid (AA) and docosahexaenoic acid (DHA) are essential for normal growth and development. Although the capacity for endogenous synthesis of LCPUFA from precursor fatty acids in preterm and term infants was thought to be limited, stable isotope studies demonstrated that both term and preterm infants have the capacity to synthesize DHA and AA.18,73 However, it remains unclear whether DHA and AA can be biosynthesized in quantities sufficient to meet the needs of these infants. In utero, LCPUFAs are supplied to the fetus across the placenta. After birth, breastfed infants receive sufficient preformed dietary LCPUFA with human milk. Although contained in human milk, DHA and AA have only recently been added to most infant formulas. Currently, most formula manufacturers have elected to add DHA and AA to their premature formulas. Multiple randomized controlled trials evaluating the addition of DHA and AA to preterm formulas have been conducted. Added DHA and AA have generally resulted in positive or neutral changes in growth, although there are some reports of a negative effect. Findings of improved visual acuity have been inconsistent. Formula supplemented with DHA and AA has produced positive changes in neurodevelopment measured in infancy in some, but not all studies. A meta-analysis demonstrated no improvement in visual acuity or neurodevelopment.79 Because studies evaluating added DHA and AA have not been conclusive, the LSRO Report assessing nutrient requirements for preterm infant formulas recommended a minimum content of zero and a maximum concentration of DHA (0.35% of total fatty acids) and AA (0.6% of total fatty acids). Cholesterol is a major component of cell membranes and a precursor in the synthesis of bile acids and some hormones. It is present in human milk in concentrations ranging from 10 to 15 mg/dL, although commercial formulas contain only trace amounts of cholesterol (approximately 1 to 2 mg/dL). The high cholesterol content of breast milk relative to formula is maintained at this level regardless of maternal diet. The groups that assessed the nutrient requirements for term and preterm infant formulas did not recommend addition of cholesterol to infant formulas because there was no convincing evidence of a beneficial short- or long-term effect of such an addition.45,59 Furthermore, there is no evidence that added cholesterol would be equivalent to the cholesterol in a human milk globule. The extremely premature neonate is born with glucose stores of only 200 kcal and loses 1% of body protein per day when provided with intravenous glucose alone. Consequently, extreme prematurity should be viewed as a nutritional emergency. A number of observational studies have described the influence of nutritional practices on growth and have found that differences in caloric and protein intake in the first weeks account for the largest difference in growth among premature infants.60 In addition, there is a growing body of data that shows the association between early nutrient intake and growth and neurodevelopmental outcomes. This section reviews the basis of recommendations for nutritional support of premature infants. Practice decisions related to provision of early nutritional support provided to ELBW infants seem to be related to the perceived severity of illness of the infant by clinicians.28 The use of standardized protocols for feeding (both parenteral and enteral) extremely premature infants can lessen variation in practice and improve outcomes. Animal studies have demonstrated a decrease in gut weight, mucosal weight, mucosal protein, DNA, disaccharide activity, and mucosal height when enteral nutrition is withheld. In a neonatal piglet model, at least 40% of total nutrient intake supplied enterally is needed to sustain normal gastrointestinal growth.15 In preterm infants, the lack of any enteral feeding leads to villous atrophy.58 A systematic review evaluated the effects of early (introduced before 96 hours of age) trophic feeding versus a comparable period of enteral fasting in VLBW infants and found no difference in feeding tolerance, growth, or necrotizing enterocolitis.13 The authors concluded that the available data cannot exclude important beneficial or harmful effects and that large randomized controlled trials are needed to evaluate the effect of early trophic feeds on important clinical outcomes in VLBW infants. Initiation of enteral nutrition is often delayed in premature infants with intrauterine growth restriction because of the concern that these infants are at an increased risk of necrotizing enterocolitis. However, a recent randomized trial evaluated early versus delayed initiation of enteral feeding for preterm growth-restricted infants and found no evidence of a difference in the incidence of necrotizing enterocolitis between groups. Consequently, the authors concluded that there was no evidence of benefit in delaying the introduction of minimal enteral feeds in preterm infants with intrauterine growth restriction beyond 24 to 48 hours of age.47a The rate at which enteral feedings should be advanced in preterm infants has also been the subject of much debate. Retrospective studies have reported an association between rapid advancement of enteral feeds and NEC. However, a systematic review evaluated the effect of slow (<24 mL/kg per day) rates of enteral feed advancement on the incidence of NEC, mortality, and other morbidities in VLBW infants and found no evidence that slow advancement of enteral feeds reduces the incidence of NEC.55 Infants who had slow rates of feeding volume advancement took longer to regain birth weight and to reach full enteral feeds. Measurement of gastric residuals is a common practice in many neonatal intensive care units to evaluate tolerance of enteral feeds despite a paucity of evidence to support the utility of gastric residuals in the diagnosis of feeding intolerance or NEC.62 In the absence of other symptoms, there is no evidence to support the discontinuation of enteral feeds based only on gastric residuals.77 Enteral feeding practices vary considerably among different centers.46 The use of evidence-based standardized feeding guidelines has been shown to improve nutritional outcomes (such as time to reach full enteral feeds), reduce number of days on parenteral nutrition, and encourage growth. In addition, standardized feeding guidelines, regardless of the content of the guideline, have been shown to reduce the incidence of necrotizing enterocolitis in premature infants.33,63 Numerous randomized clinical trials of amino acid dose and advancement strategy to evaluate short-term tolerance, protein balance, and safety have been conducted in premature infants. Despite differences in study populations and composition of amino acid solutions, all studies demonstrated positive nitrogen balance in response to parenteral amino acids and improved protein balance with higher amino acid intake.41,43,71,93,95 It is also important to point out that positive nitrogen balance was found despite low total caloric intake (approximately 50 kcal/kg per day). Consequently, the initial goal of limiting catabolism and preserving endogenous protein stores in premature infants can be accomplished with provision of as little as 1.0 to 1.5 g/kg per day of intravenous amino acids, whereas delivery of 3 g/kg per day of amino acids will result in net protein gain that approximates that of the reference fetus (see Figure 43-1). Stable isotope techniques have also been used to evaluate the effect of amino acids on protein metabolism in premature infants. In these studies, stable isotope tracers of one or more essential amino acids are used to reflect whole body protein kinetics. Rivera and colleagues found that administration of 1.5 g/kg per day of amino acids (Aminosyn-PF with cysteine added) beginning on the first day of life improved protein balance as a result of increased protein synthesis (as reflected by leucine kinetics).71 Using similar techniques, van den Akker and colleagues demonstrated that protein anabolism produced by administration of early amino acids is accomplished through an increase in protein synthesis and not from a decrease in proteolysis (protein breakdown).92 Other investigators have assessed the safety and efficacy of different doses of amino acids. Thureen and colleagues conducted a randomized trial of low (1 g/kg per day) versus high (3 g/kg per day) amino acid intake in infants with extremely low birth weights immediately after birth.88 The higher amino acid intake produced significantly greater protein accretion. In an open-label trial, te Braake and colleagues randomized VLBW infants to two different parenteral amino acid regimens.87 Infants in the intervention group received 2.4 g/kg per day of amino acids starting immediately after birth. Infants in the control group received glucose alone on the first day of life, with a stepwise increase in amino acid intake thereafter (1.2 g/kg on day 2 and 2.4 g/kg on days 3 and 4). Infants who received amino acids on the first day of life were found to have positive nitrogen balance without any major adverse effects, whereas infants in the control group were in negative nitrogen balance on day 2. Ibrahim and colleagues evaluated an even higher initial dose of intravenous amino acids, randomizing VLBW premature infants to either 2 g/kg or 3.5 g/kg per day of intravenous amino acids on the first day of life. Similar to the studies discussed previously, the higher amino acid dose resulted in greater improvement in nitrogen balance without evidence of adverse effects.41 The safety of administration of early intravenous amino acids, particularly for premature infants, has been established in a variety of studies. Normal plasma amino acid concentrations have been reported using TrophAmine.39 Rivera and colleagues studied infants with very low birth weights given amino acids in the first days of life and found no abnormal elevations of plasma amino acids, BUN, or ammonia.72 Thureen and colleagues found that 3 g/kg per day of early amino acids appears to be as safe as 1 g/kg per day, based on BUN and plasma aminograms as indicators of acute amino acid toxicity.61,88 Although most studies have not demonstrated any relationship between amino acid intake and BUN in the first days of life, two studies reported increased BUN levels in infants receiving higher amino acid intakes at 7 days of age.12,20 As mentioned earlier, a significant proportion of the amino acids supplied to the developing fetus are oxidized and serve as a significant energy source for the fetus. Urea production is a byproduct of amino acid oxidation. In premature infants, rates of urea production are higher than in term neonates and adults, consistent with high rates of protein turnover and oxidation. Some investigators have even suggested that azotemia might be evidence of the effective utilization of amino acids as an energy supply rather than of protein intolerance, but this contention remains unproven. There are no data to support the need to advance amino acid intake slowly. Although the initial goal of providing intravenous amino acids to premature infants to limit catabolism and preserve endogenous protein stores can be accomplished even if total caloric intake is low, ultimately, both protein and energy must be supplied in quantities sufficient to support optimal growth. Protein quantity is the primary determinant of protein accretion. This is true regardless of whether parenteral nutrition is used exclusively or as a bridge to full enteral feedings. In a series of studies, Zlotkin and colleagues evaluated the effect of intravenous energy and nitrogen intake on nitrogen retention.104 At constant nitrogen intake, increasing nonprotein energy intake from 50 to 80 kcal/kg per day resulted in increased nitrogen retention and weight gain. However, at low energy intake (~50 kcal/kg per day), increasing nitrogen intake from 494 to 655 mg/kg per day (3 to 4 g/kg of amino acids per day) had no effect on nitrogen retention or weight gain. However, at higher energy intakes (~80 kcal/kg per day), the same increase in nitrogen intake resulted in a significant increase in the rate of both nitrogen retention and weight gain. Many conditions and interventions commonly encountered in extremely premature infants are known to increase protein requirements. Underlying disease states such as sepsis or surgical stress increase catabolism and can negatively impact protein accretion. In addition medications such as systemic steroids, fentanyl, and insulin can also impact protein accretion. Dexamethasone has been shown to increase protein catabolism by increasing protein oxidation and proteolysis, resulting in decreased accretion of protein.94 The newest solutions include modifications of crystalline amino acids for use in pediatric patients. The currently available solutions include modifications of crystalline amino acids for use in pediatric and neonatal patients (Table 43-4). TrophAmine was originally formulated to match plasma amino acid concentrations of healthy term, breastfed infants; Premasol is identical in composition to TrophAmine. The composition of Primene, available outside the United States, was derived from fetal and neonatal cord blood concentrations. Both TrophAmine and Premasol supply a mixture of L-tyrosine and N-acetyltyrosine. The bioavailability of N-acetyltyrosine, however, has been questioned. Neither Aminosyn-PF nor Primene supplies a substantial amount of tyrosine. Cysteine is not supplied by most amino acid solutions because it is not stable for long periods of time in solution. However, cysteine hydrochloride can be added during the compounding process just prior to delivery of the solution. TABLE 43-4 Composition of Parenteral Amino Acid Solutions* *Amino acid concentration in mg/dL; all amino acid mixtures shown are 10% solutions. Tyrosine is not present in appreciable amounts in currently available amino acid solutions because of its low solubility. Snyderman found lower rates of weight gain, nitrogen retention, and plasma concentrations of tyrosine in premature infants given a tyrosine-deficient diet.80 Tyrosine is synthesized endogenously from phenylalanine by phenylalanine hydroxylase. The activity of this enzyme in premature infants was thought to be inadequate for growth and nitrogen retention without tyrosine supplements. However, stable isotope studies have demonstrated active phenylalanine hydroxylation in very premature (26 weeks) and premature (32 weeks) infants.21,24 Therefore, in the strictest sense, tyrosine is not an essential amino acid. However, it remains unclear whether enough tyrosine can be endogenously produced from phenylalanine in premature infants to support normal rates of protein accretion. N-acetyl tyrosine, although currently added to TrophAmine, is not highly bioavailable. Nonetheless, several studies provide indirect evidence that N-acetyl tyrosine improves protein accretion in preterm infants.36,38 In addition, it is unclear whether premature infants can adequately catabolize tyrosine via oxidation by the enzymes tyrosine aminotransferase and 4-hydroxyphenylpyruvate dioxygenase. Inability to catabolize tyrosine can lead to transient neonatal tyrosinemia. Further studies are needed to better define premature infants’ ability to catabolize tyrosine and to determine whether an alternative source of tyrosine is needed in parenteral amino acid solutions. Cysteine may be a conditionally essential amino acid for premature infants, but is not contained in currently available amino acid solutions. Some studies have shown that the fetal liver lacks the enzyme system to convert methionine into cysteine and that infants on a cysteine-free diet demonstrate impaired growth and low plasma cysteine levels. Other studies have demonstrated that there is enough cystathionase in extrahepatic tissues of the fetus and premature infant to synthesize cysteine when an adequate amount of methionine is provided. Studies using stable isotope techniques have demonstrated active endogenous cysteine synthesis in low birth weight infants.76 Nevertheless, there is evidence to support that when cysteine hydrochloride supplements are added to parenteral nutrition, nitrogen retention is improved in premature infants.81 Further, the addition of cysteine hydrochloride improves the solubility of calcium and phosphorus in parenteral nutrition solutions. However, it is important to note that cysteine hydrochloride supplements can produce metabolic acidosis unless appropriately buffered with acetate. Glutamine is one of the most abundant amino acids in both plasma and human milk, yet it is not supplied by currently available amino acid solutions because glutamine is unstable in aqueous solution. Glutamine is a major energy substrate for small intestinal mucosa, as proved by a high glutamine uptake from the lumen and from arterial blood during the newborn period in rats. Adding glutamine to the parenteral nutrition (PN) solutions of animals prevents atrophy of small intestinal mucosa and smooth muscle, improves the gut immune function, and reduces the incidence of fatty infiltration of the liver. Several studies suggest that parenteral glutamine supplementation is of benefit in selected populations of critically ill adults. However, a large, multicenter, randomized clinical trial of parenteral glutamine supplementation found that parenteral glutamine supplementation did not decrease mortality or the incidence of late-onset sepsis in ELBW infants.65
Nutrient Requirements and Provision of Nutritional Support in the Premature Neonate
Growth and Neurodevelopmental Outcomes
Nutrient Requirements
Protein and Amino Acid Requirements
Weight <1200 g
Weight >1200 g
g/kg/d
g/100 kcal
g/kg/d
g/100 kcal
Ziegler103
4.0
3.7
3.6
2.8
Kashyap & Heird43
—
—
3.0
2.5
Tsang69
3.8-4.2
3.3
3.4-3.6
2.8
LSRO45
3.4-4.3
2.5-3.6
3.4-4.3
2.5-3.6
ESPGHAN3
4.0-4.5
3.6-4.1
3.5-4.0
3.2-3.6
Body Weight (g)
Protein (g/kg/d)
Energy (kcal/kg/d)
Protein/Energy (g/100 kcal)
500-700
4.0
105
3.8
700-900
4.0
108
3.7
900-1200
4.0
119
3.4
1200-1500
3.9
125
3.1
1500-1800
3.6
128
2.8
1800-2200
3.4
131
2.6
Energy and Carbohydrate Requirements
Fetal Glucose Metabolism
Neonatal Glucose Homeostasis
Energy Expenditure
Energy Expenditure
kcal/kg/d
Resting metabolic rate
40-60
Activity
0-5
Thermoregulation
0-5
Synthesis/energy cost of growth
15
Energy stored
20-30
Energy excreted
15
Total energy requirement (estimated)
90-120
Carbohydrates
Recommended Energy Intake
Lipid Requirements
Provision of Nutritional Support
Initiation and Advancement of Enteral Feeds
Minimal Enteral Feeding
Rate of Advancement of Enteral Feeds
Gastric Residuals
Standardized Feeding Guidelines
Evidence Supporting Early Parenteral Amino Acids
Intravenous Amino Acid Mixtures
Aminosyn-PF
TrophAmine Premasol
Primene
Histidine
312
480
380
Isoleucine
760
820
670
Leucine
1200
1400
1000
Lysine
677
820
1100
Methionine
180
340
240
Phenylalanine
427
480
420
Threonine
512
420
370
Tryptophan
180
200
200
Valine
673
780
760
Alanine
698
540
800
Arginine
1227
1200
840
Proline
812
680
300
Serine
495
380
400
Taurine
70
25
60
Tyrosine
44
240†
45
Glycine
385
360
400
Cysteine
—
<16
189
Glutamic Acid
820
500
1000
Aspartic Acid
527
320
600
Stay updated, free articles. Join our Telegram channel
Full access? Get Clinical Tree
Nutrient Requirements and Provision of Nutritional Support in the Premature Neonate
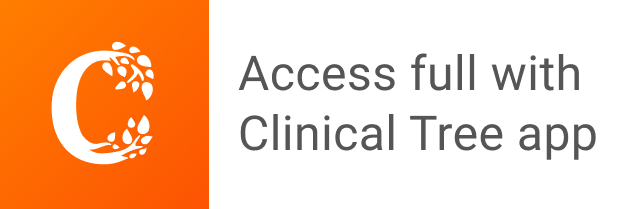