Metabolism
Patrick J. Javid
Thomas Jaksic
Department of Surgery, Children’s Hospital Boston, Boston, Massachusetts 02115.
Harvard Medical School, Children’s Hospital Boston, Boston, Massachusetts 02115.
The metabolism of the child with critical illness, traumatic injury, or postsurgery is altered in a predictable fashion. Although this response is generally beneficial in the short term, it can become deleterious if illness persists. The associated changes are chiefly catabolic in nature and involve the dramatic mobilization of host substrate to facilitate the healing process. The consequences of such metabolic alterations are especially significant in the child as the baseline requirements for growth and development are increased, while tissue stores are limited. Thus, the critically ill child is at increased risk for morbidity and mortality if nutritional support is inadequate or not instituted promptly.
The fundamental basis for the metabolic response to injury was first described by Sir David Cuthbertson in the 1930s (1). He was the first investigator to realize the role of whole-body protein catabolism in the systemic response to injury. He surmised that the metabolic state following injury progressed through two stages: an initial hypometabolic ebb phase, followed quickly by a prolonged increase in overall metabolic rate termed the flow phase (2). It was later determined that this increased degree of tissue breakdown was ameliorated, but not eliminated, by the provision of dietary protein (3). In the 1950s, Dr. Francis D. Moore carefully elucidated the hormonal and substrate mechanisms responsible for the body’s response to surgery (4). Finally, with the advent of techniques allowing the identification of molecular structures, numerous small peptide mediators called cytokines were discovered as the mechanistic agents of the unified inflammatory and metabolic stress response to injury.
Given the unique growth patterns and energy requirements of the neonate and child, it is no surprise that certain aspects of the pediatric metabolic response to illness differ from those of the adult. Research into the stress response has demonstrated that, although children and adults share similar qualitative metabolic changes, significant quantitative differences in substrate turnover and energy metabolism exist.
BASIC NUTRITIONAL BIOCHEMISTRY
Substrate Metabolism
Children and adults rely on the release of energy from ingested nutrients or established body stores to fuel the work of the human body. In this way, the energy derived from the degradation of large substrate molecules is used to synthesize high-energy phosphate bonds in the form of adenosine triphosphate (ATP). The bulk of energy metabolism in the human is performed in the aerobic setting, although anaerobic pathways exist and indeed are accentuated in the setting of stress or disease. The hydrolytic cleavage of a phosphate group from ATP, thereby producing adenosine diphosphate, provides much of the energy needed for cellular reactions.
The major sources of energy to fuel the processes of work are carbohydrate, lipid, and protein. These substrates are degraded by the body into the currency of energy metabolism: glucose, fatty acids, and amino acids. For most tissues, fatty acids are the major source for oxidative energy production. However, glucose is of great importance because it is the preferred energy source for cells in the central nervous system, renal medulla, and erythrocytes. These tissues cannot take up fatty acids from the systemic circulation, and therefore rely on glucose or ketone bodies during starvation. Pathways exist to synthesize glucose from the breakdown of amino acid and lipid substrates, a process that is accelerated in critical illness. In general, the breakdown and synthesis of glucose, lipid, and protein are tightly controlled by neural, hormonal, and allosteric regulation.
In the fasting state, the body mobilizes host substrate to provide energy in the form of glucose for the continuation of cellular work. Glycogen, the storage form of glucose
found in liver and skeletal muscle, is the first substrate to be broken down for glucose production. Glycogen stores are limited in both the adult and child, and are effectively depleted within 24 hours. At this point, host protein stores, and to a lesser extent the glycerol backbone of triglycerides, must be mobilized for the continued supply of glucose. The glucose that is produced may be used in a variety of ways: (1) transported to the brain, kidney, and erythrocyte for cellular energy; (2) catabolized via glycolysis and the citric acid cycle, and ultimately used in oxidative phosphorylation for ATP production; or (3) in the anaerobic setting, degraded via glycolysis for limited energy and ATP production. Glycolysis in the anaerobic setting is responsible for the production of lactic acid from pyruvate. In the Cori cycle, lactate produced from peripheral tissues is transported to the liver, where it can be converted back to pyruvate and used to produce glucose. In this way, during anaerobic metabolism, the liver can continue to supply extrahepatic tissues with glucose while peripheral tissue can use glycolysis for a limited energy supply. The yield from the catabolism of one molecule of glucose from oxidative phosphorylation is 38 molecules of ATP versus only 2 molecules of ATP in glycolysis. Clearly, aerobic oxidation of glucose is the preferred pathway for energy production in the human.
found in liver and skeletal muscle, is the first substrate to be broken down for glucose production. Glycogen stores are limited in both the adult and child, and are effectively depleted within 24 hours. At this point, host protein stores, and to a lesser extent the glycerol backbone of triglycerides, must be mobilized for the continued supply of glucose. The glucose that is produced may be used in a variety of ways: (1) transported to the brain, kidney, and erythrocyte for cellular energy; (2) catabolized via glycolysis and the citric acid cycle, and ultimately used in oxidative phosphorylation for ATP production; or (3) in the anaerobic setting, degraded via glycolysis for limited energy and ATP production. Glycolysis in the anaerobic setting is responsible for the production of lactic acid from pyruvate. In the Cori cycle, lactate produced from peripheral tissues is transported to the liver, where it can be converted back to pyruvate and used to produce glucose. In this way, during anaerobic metabolism, the liver can continue to supply extrahepatic tissues with glucose while peripheral tissue can use glycolysis for a limited energy supply. The yield from the catabolism of one molecule of glucose from oxidative phosphorylation is 38 molecules of ATP versus only 2 molecules of ATP in glycolysis. Clearly, aerobic oxidation of glucose is the preferred pathway for energy production in the human.
When glycogen has been depleted, lipid is the next substrate to be mobilized for energy production. Triglycerides stored in the cell are broken down and transported in the form of fatty acids to the liver, muscle, and heart. Here, individual fatty acids are degraded in the process of β-oxidation, which produces one acetyl-coenzyme A (acetyl-CoA) molecule for every two carbon groups in the fatty acid. Acetyl-CoA can then be oxidized further through the citric acid cycle and ultimately used to produce ATP via oxidative phosphorylation. Acteyl-CoA in mammalian tissue cannot undergo conversion to glucose. The glycerol backbone of the triglyceride is converted to an intermediate in glycolysis and can also be used for energy production or gluconeogenesis.
In settings of extreme fasting or uncontrolled diabetes mellitus, the metabolic pathways are regulated to prioritize the production of glucose for fuel to extrahepatic tissues. The accelerated production of glucose depletes the hepatocyte of needed intermediates in the citric acid cycle. When this occurs, the acetyl-CoA generated from the breakdown of fatty acids cannot enter the citric acid cycle and instead forms the ketone bodies, acetoacetate and β-hydroxybutyrate, through a nonenzymatic condensation reaction. These ketone bodies are released by the liver to extrahepatic tissues, including skeletal muscle and the brain, where they can be used for energy production. In these peripheral tissues where the citric acid cycle remains active, ketone bodies enter the citric acid cycle for ATP production. The system of ketone body formation allows continued oxidation of fatty acids in the liver for the provision of energy to peripheral tissue when glucose is not available. During surgical illness, insulin levels are elevated and hence ketone body formation is relatively inhibited. Thus, ketone bodies do not significantly supplant the need for glucose in surgical patients.
Under normal conditions, protein stores are not a primary substrate for the generation of energy in the human body. Rather, protein serves as the body’s major component for synthesis and structure. Proteins, and their constituent amino acids, however, are never static and exhibit a “turnover” due to constant degradation and synthesis. In settings of critical illness and injury, protein breakdown is accelerated significantly. When amino acids must be substituted as a substrate for energy production, the body gradually loses its structural protein stores. Net protein loss in critical illness must be avoided in order to maintain the host’s capacity to heal wounds and avoid infection. In children, protein loss halts growth and normal development.
When amino acid catabolism occurs, the initial step involves removal of the toxic amino group (NH3) from the amino acid. Through transamination, the NH3 is transferred to α-ketoglutarate, thereby producing glutamate. The addition of an additional amino group converts glutamate to glutamine, which is then transported to the liver. Here, the amino groups are removed and the ammonia detoxified to urea via the urea cycle. Alternatively, in muscle tissue the amino group can be transferred to pyruvate, forming the amino acid alanine. When alanine is detoxified in the liver, pyruvate is thus reformed and can be converted into glucose via gluconeogenesis. The transport of alanine between peripheral tissue and the liver for ammonia detoxification and glucose generation is termed the glucose-alanine cycle. Hence, the transport amino acid systems involving glutamine and alanine provide carbon backbones for gluconeogenesis in the liver while they facilitate the detoxification of ammonia by the urea cycle.
In the postprandial state, the glucose, amino acids, and fatty acids absorbed from the small intestine are distributed by the liver for appropriate energy metabolism. The majority of fatty acids are packaged by the enterocyte into chylomicrons and excreted into the lymphatic system. These lipid molecules will be stored as triglycerides or phospholipids in adipose tissue. The remaining medium-chain fatty acids are transported to the hepatocyte with glucose and amino acids via the portal circulation. The liver oxidizes fatty acids to form acetyl-CoA, which can enter the citric acid cycle and proceed to oxidative phosphorylation. Tissues that cannot take up fatty acids from the systemic circulation—the brain, renal medulla, and red blood cells—rely primarily on glucose for energy needs.
Glucose can be shunted to several different pathways by the liver following a meal. If blood sugar is low, some glucose will be released into the systemic circulation to maintain glucose homeostasis. Alternatively, glucose
can be phosphorylated to glucose-6-phosphate, an intermediate of multiple pathways. Glucose-6-phosphate can be (1) used for glycogen production to store excess glucose, (2) converted to acetyl-CoA by glycolysis and subsequent energy production via the citric acid cycle and oxidative phosphorylation, (3) converted to acetyl-CoA and shunted toward lipid biosynthesis, or (4) oxidized in the pentose phosphate pathway for ultimate conversion to steroid precursors and nucleotides.
can be phosphorylated to glucose-6-phosphate, an intermediate of multiple pathways. Glucose-6-phosphate can be (1) used for glycogen production to store excess glucose, (2) converted to acetyl-CoA by glycolysis and subsequent energy production via the citric acid cycle and oxidative phosphorylation, (3) converted to acetyl-CoA and shunted toward lipid biosynthesis, or (4) oxidized in the pentose phosphate pathway for ultimate conversion to steroid precursors and nucleotides.
Amino acids in the hepatocyte can be used for the synthesis of both hepatic and extrahepatic proteins, including structural proteins and synthetic enzymes. Extra supplies of amino acid in the hepatocyte are catabolized to citric acid precursors and degraded for oxidative energy production.
The process of substrate metabolism is similar in adults and children, although noteworthy differences exist. Neonates in particular have a high demand for glucose, which may be secondary to their increased brain-to-body mass ratio. In addition, glycogen stores are relatively depleted in the neonate, particularly in the preterm infant (5). Clinically, short periods of fasting can predispose the newborn infant to hypoglycemia. Thus, in these patients, the body must turn to the breakdown of lipid and protein stores earlier in the process of substrate metabolism to provide an effective energy source. However, as the majority of polyunsaturated fatty acids accumulate toward the end of pregnancy, the premature child has a lower proportion of lipid stores. Neonates also have decreased lipid stores as compared with the adult, thereby rendering lipid less useful as a potential fuel source (6). Taken together, the neonate’s increased energy needs and limited substrate stores mandate early nutritional support in settings of critical illness.
Measurement of Energy Requirements
Total energy requirement for all patients includes resting energy expenditure, energy allotted to physical activity, and diet-induced thermogenesis. By definition, resting energy expenditure encompasses the body’s energy requirement for growth. Physical activity, which is low in the postoperative period or during severe illness, and diet-induced thermogenesis are of reduced significance in the surgical patient. Knowledge of resting energy expenditure and its changes following critical illness or operation is important because it directly impacts energy requirements.
Resting energy expenditure can be measured in a variety of ways, including direct and indirect calorimetry, as well as stable isotopic techniques. The direct method measures the heat released by a subject at rest and during various activities. This method is based on the principle that all energy is eventually converted to heat. In practice, the subject is placed in a thermally isolated chamber with sensitive temperature measurement over a given period of time (7). A change in temperature of the chamber is used to calculate the energy discharged, and therefore fuel oxidized, during the time period. Although precise, this method is not practical for most pediatric or ill patients (8).
Indirect calorimetry, the most commonly used technique, estimates energy production based on quantities of O2 consumption and CO2 production by the body during a specific time interval. The device measures the VO2 (volume of O2 consumed), VCO2 (volume of CO2 produced), and a correction factor based on urinary nitrogen excretion to calculate the overall rate of energy production (9). Usually, a “metabolic cart”—composed of a leak-free system with a microcomputer-controlled gas exchange measurement device—is used in conjunction with a 24-hour urine collection. Indirect calorimetry provides a measurement of the overall respiratory quotient (RQ), defined as the ratio of CO2 produced to O2 consumed. Although this method is often useful in the adult patient, its accuracy is impaired in pediatric patients with uncuffed endotracheal tubes, babies who may not be breathing calmly, or in those infants who require extracorporeal membrane oxygenation (ECMO) (10).
Recently, two independent nonradioactive stable isotope techniques have been developed to more accurately measure resting energy expenditure in the pediatric population. The infusion of a small amount of stable 13C-labeled bicarbonate allows the calculation of energy expenditure solely on the basis of infusion rate and the ratio of labeled to unlabeled tracer in expired breath samples (11). Similarly, an infusion of stable isotopic doubly labeled water (2H218O) requires only an assessment of hydrogen and oxygen enrichment that can be measured in serial urine samples (12). Neither technique requires sampling of blood. Both the labeled bicarbonate and the doubly labeled water techniques have been validated and effectively studied in previous investigations in neonates (12,13).
Energy Reserves and Requirements
The neonate and child differ significantly from the adult patient in the proportion of available metabolic reserves. Table 8-1 outlines the macronutrient reserves of the
neonate, child, and adult in percentage of total body weight (14,15,16). Carbohydrate stores are limited in all age groups and afford only acute provisions when necessary. Lipid reserves, an important and efficient source of energy, are reduced in the neonate as compared with the adult and gradually increase with age. The most striking difference between the adult and pediatric patient is the quantity of stored protein. The protein reserve of the adult is nearly twice that of the neonate.
neonate, child, and adult in percentage of total body weight (14,15,16). Carbohydrate stores are limited in all age groups and afford only acute provisions when necessary. Lipid reserves, an important and efficient source of energy, are reduced in the neonate as compared with the adult and gradually increase with age. The most striking difference between the adult and pediatric patient is the quantity of stored protein. The protein reserve of the adult is nearly twice that of the neonate.
TABLE 8-1 Body Composition of Neonates, Children, and Nonobese Adults as a Percentage of Total Body Weight. | ||||||||||||||||
---|---|---|---|---|---|---|---|---|---|---|---|---|---|---|---|---|
|
Along with lower metabolic reserves, neonates and children share much higher baseline energy requirements. Studies have consistently demonstrated that the resting energy expenditure for neonates is two to three times that of adults when standardized for body weight (17,18,19). Clearly, the child’s rapid growth and cellular differentiation heavily influence the magnitude of the corresponding energy metabolism. In addition, the relationship between increased energy expenditure and decreased body weight is conserved throughout mammalian species. This may be related to the increased body surface area and possibly a higher brain-to-body mass ratio.
Basic protein and energy requirements for the neonate, child, and adult based on recommendations by the National Academy of Sciences are compared in Table 8-2 (20). The protein needs of the neonate are almost three times that of the healthy adult. In premature infants, a minimum protein allotment of 2.8 g per kg per day is required to maintain in utero growth rates (21). Thus, with both increased metabolic demand and limited substrate reserve, the child has the significant potential for profound nutritional deficiency in settings of critical illness and injury.
TABLE 8-2 Recommended Energy and Protein Requirements in Healthy Humans of Various Ages. | ||||||||||||
---|---|---|---|---|---|---|---|---|---|---|---|---|
|
NUTRIENT METABOLISM IN CRITICAL ILLNESS
Figure 8-1 illustrates the changes in metabolism that occur in children and adults with the onset of critical illness. Turnover rates of protein, carbohydrate, and lipid substrates increase dramatically, and net protein breakdown is accentuated. In general, muscle protein is degraded and the amino acid by-products are transported to the liver. Once in the liver, the amino acids are used for the production of acutely needed inflammatory proteins. The remaining amino acid moieties are shunted into the gluconeogenic pathway, and the ammonia end product is detoxified to urea. The glucose thus produced is used for
energy in a diverse array of tissues. The understanding of the metabolic changes associated with critical illness in the young child is paramount in the implementation of effective nutritional support for these patients.
energy in a diverse array of tissues. The understanding of the metabolic changes associated with critical illness in the young child is paramount in the implementation of effective nutritional support for these patients.
Protein Metabolism
Amino acids are the building blocks necessary for tissue growth and repair, and 98% of amino acids reside in protein at a given time. However, proteins are never static and are continually degraded and synthesized in the process of protein turnover. High protein turnover allows the body maximal flexibility in meeting ever-changing physiologic demands. The process is energy dependent, however, and may contribute to altered resting energy expenditures in critically ill patients.
Infants are known to have higher rates of protein turnover than adults. The healthy newborn has a protein turnover of 6 to 12 g per kg per day compared with 3.5 g per kg per day in adults (22). Premature and low birth weight infants have even greater rates of protein turnover (22,23). At the same time, infants must maintain a positive protein balance to afford growth and development, whereas neutral protein balance will suffice in the healthy adult.
In metabolically stressed patients, such as children with severe burn injury, neonates with cardiorespiratory failure requiring ECMO, or children with diagnosed malignancy, protein turnover is doubled when compared with normal subjects (13,24,25). A more recent study of critically ill infants and children found an 80% increase in protein turnover, which correlated with duration of the critical illness (26). This process involves a redistribution of amino acids away from skeletal muscle to the liver, wound, and tissues taking part in the inflammatory response. In this way, acutely needed enzymes, serum proteins, and glucose are synthesized from amino acids. Evidence for this redistribution is found in the marked rise of hepatically derived acute phase proteins (i.e., C-reactive protein, fibrinogen, transferrin, α-1-acid glycoprotein) and the concomitant decrease in transport proteins, including albumin- and retinol-binding protein.
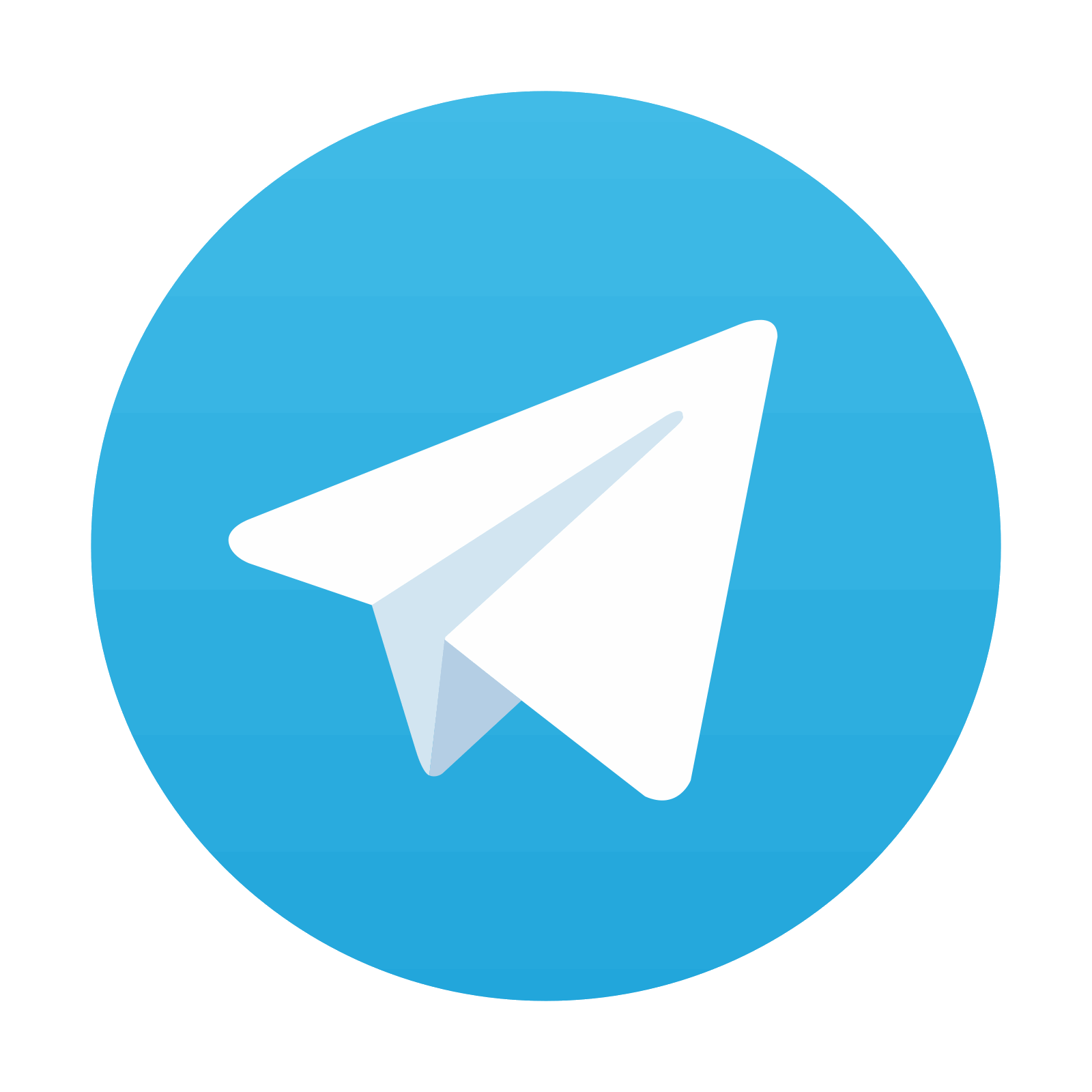
Stay updated, free articles. Join our Telegram channel
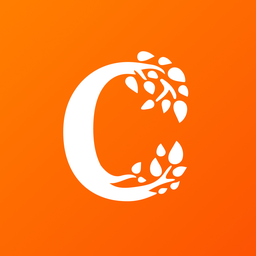
Full access? Get Clinical Tree
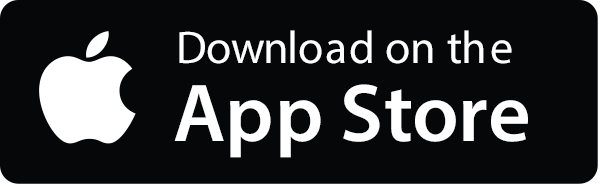
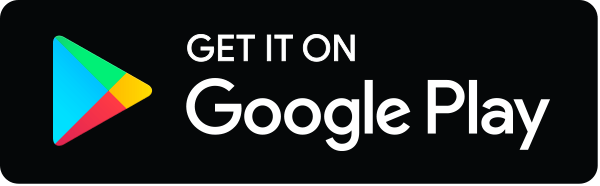