and Peter C. Rimensberger8
(7)
Pediatric Intensive Care Unit, University Medical Center Utrecht, 85090, 3508 AB Utrecht, The Netherlands
(8)
Department of Pediatrics, Service of Neonatology and Pediatric Intensive Care, University Hospital of Geneva, >CH-1211 Geneva 14, Switzerland
Educational Aims
-
Understand essential mechanics of HFOV.
-
Understand causes of ventilator-induced lung injury.
-
Be able to translate this knowledge into ventilation strategies that aim to minimize deleterious effects of mechanical ventilation.
-
Know the characteristics of HFOV that help to prevent ventilator-induced lung injury.
-
Know the evidence for choosing HFOV as an elective therapy.
-
Know the evidence for choosing HFOV as a rescue therapy.
-
Be able to assess which patients will benefit from HFOV based on theory and evidence.
-
Know which patients are eligible for HFOV in the neonatal intensive care unit.
-
Know which patients are eligible for HFOV in the pediatric intensive care unit.
19.1.1.1 Introduction
Critical care physicians facing patients that require invasive mechanical ventilation are confronted with the questions what type of ventilator and what ventilator strategy is most appropriate for this particular patient. Mechanical ventilation is initiated to bridge a gap to recovery or to supply chronic support. Failure of mechanical ventilation can arise through inability to deliver adequate gas exchange or through resulting complications, like pneumothorax. Although mechanical ventilation is meant to support respiratory function, mechanical ventilation can also result in pulmonary damage and contribute to increased risk of mortality. Therefore, the selection of the ventilator type and strategy should be guided by the capacity to achieve adequate gas exchange and, in the meanwhile, to prevent lung injury.
The two basic types of ventilators that can be considered to achieve these goals are the conventional mechanical ventilator or one of several types of high-frequency ventilators. In this section we will focus on high-frequency oscillatory ventilators (HFOV). Using conventional mechanical ventilation (CMV), tidal volumes at more or less physiological breathing rates are delivered, while with high-frequency oscillatory ventilation (HFOV), respiratory rates are employed far above the physiological range.
Up till now CMV is standard of care. The indications to use HFOV can be classified into two categories: (1) rescue indications, i.e., when CMV fails to sustain adequate gas exchange or leads to major complications, or (2) elective indications, i.e., in which it is anticipated that use of HFOV improves overall prognosis compared to HFOV. Treatment failure of CMV can reveal itself by insufficient carbon dioxide elimination despite high tidal volumes or impaired oxygenation despite high fractional inspiratory oxygen and high mean airway pressure. Failure to support gas exchange can be addressed by providing additional measures like nitric oxide inhalation or prone positioning. Alternative therapies can be considered next, like HFOV or ultimately lung replacement therapies like extracorporeal membrane oxygenation. Ventilation-associated complications like air leak syndromes or cardiovascular compromise can also prompt for these alternative therapies.
To understand the rationale for choosing HFOV as an alternative therapy for HFOV, two important aspects of mechanical ventilation have to be recognized. First, over the past decades, it has become apparent that mechanical ventilation can have deleterious effects both locally, resulting in lung damage, and systematically, resulting in increased mortality. Secondly, the effects of mechanical ventilation are more importantly determined by ventilation strategy than by the type of ventilator that is used. Optimal ventilation strategy is determined by patient features and by disease characteristics. The type of ventilator can enable or constraint application of ventilation strategy.
19.1.1.2 Educational Aims
In this chapter, we will discuss indications for HFOV. Why and when should HFOV be considered and in which neonatal and pediatric patients? The answers to those questions are closely connected to theories and evidence concerning ventilator-induced lung injury and lung protective ventilation strategies to prevent lung injury. Therefore, it is important to understand the concepts of ventilator-induced lung injury and how this translates to lung protective ventilation strategies.
The rationale for choosing HFOV instead of CMV will focus on the differences between these two modes of ventilation that will facilitate use of a lung protective ventilation strategy.
19.1.1.3 Pathophysiological Rationale for Choosing HFOV
19.1.1.3.1 What Is HFOV?
19.1.1.3.1.1 HFOV Characteristics
HFOV can be described by the following characteristics: (1) ventilation at high respiratory rate, generally 3–25 Hz; (2) use of a continuous distending airway pressure; (3) superimposed ventilation with small tidal volumes, typically less than anatomical dead space; and (4), specifically for HFOV, an active expiration phase. In other types of high-frequency ventilation, the expiration phase is mainly passive.
HFOV employs a piston or diaphragm to oscillate a bias flow of gas (between 10 and 40 l, according to patient size and demands) to produce alternating positive- and negative-pressure swings. A continuous distending pressure is generated by a low-pass filter that acts as a resistor. HFOV is unique in its active expiration phase created by the backward movement of the diaphragm that generates a negative pressure.
19.1.1.3.1.2 HFOV Operation and Gas Exchange
Operator-selected parameters include adjustment of the following: mean airway pressure (continuous distending pressure), frequency, inspiratory time, pressure amplitude, bias flow, and oxygen concentration of inspired gas. Like in CMV, to improve oxygenation, mean airway pressure is increased. However, in HVOV, regulation of oxygenation is uncoupled from carbon dioxide elimination, i.e., adjustment of the mean airway pressure does not necessarily affect carbon dioxide elimination. Moreover, by decreasing frequency, unlike in CMV, carbon dioxide elimination is increased and vice versa. Inspiratory time can be adjusted to improve oxygenation but is typically set at 33 %. The amplitude can be adjusted upward and downward to increase and decrease carbon dioxide elimination, respectively. Bias flow can be adjusted to maintain mean airway pressure and depends on patient size and demands.
During CMV, carbon dioxide removal is accomplished by bulk convection and is directly related to minute ventilation, the product of tidal volume and frequency. HFOV achieves gas transport with tidal volumes that are below anatomic dead space; therefore, carbon dioxide must be “washed out” by additional mechanisms. In HFOV, carbon dioxide elimination is directly related to ventilatory frequency and the square of delivered volume that is a function of amplitude. Because of the technical characteristics of the device, the delivered volume is inversely related to frequency. Frequency controls the time allowed for the piston to move, i.e., the lower the frequency, the greater the volume displacement and vice versa. It is recommended to set the amplitude at the highest possible pressure and adjust the frequency accordingly. This will result in the highest possible frequency as well, as frequency and pressure amplitude have an inverse effect on carbon dioxide elimination (Fessler et al. 2007; Froese 1997; Hager et al. 2007).
19.1.1.3.1.3 Other Types of High-Frequency Ventilation
There are four major types of high-frequency ventilation: (1) high-frequency positive-pressure ventilation (frequency 1–2.5 Hz), (2) high-frequency jet ventilation (frequency 1.7–10 Hz), (3) high-frequency flow interruption (6–15 Hz), and (4) high-frequency oscillatory ventilation (HFOV, frequency 3–15 Hz). High-frequency positive-pressure ventilation and high-frequency jet ventilation promote gas exchange in a more conventional way, i.e., tidal volumes approximate or exceed dead space volume, and expiration is passive. In contrast, in HFOV tidal volumes are generated that are less than dead space and expiration phase is active. It has been estimated that tidal volumes delivered during HFOV are about 1–4 mL/kg, depending on frequency (Sedeek et al. 2003). HFOV is now the most commonly used method of high-frequency ventilation with a good and long-standing experience in neonatal and pediatric intensive care (Rimensberger 2003).
19.1.1.3.2 What Factors Cause Ventilator-Induced Lung Injury?
The injurious effects of mechanical ventilation on lung tissue have been designated as ventilator-induced lung injury (Dreyfuss and Saumon 1998; Pingleton 1988). Ventilator-induced lung injury is a concept that has been extensively investigated in animal experimental studies. It represents a complex disorder that is caused by a number of ventilator-related factors. These main explanatory mechanisms have been called barotrauma, volutrauma, atelectotrauma, and biotrauma (Fig. 19.1) (Dreyfuss and Saumon 1992, 1998; Parker et al. 1993).
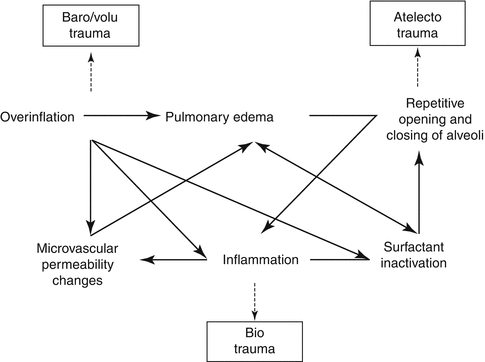
Fig. 19.1
Pathophysiological mechanisms of ventilator-induced lung injury. Three major mechanisms lead to ventilator-induced lung injury: (1) barotrauma/volutrauma, (2) atelectotrauma, and (3) biotrauma. There is a reinforcing interaction between the different mechanisms leading to a number of vicious circles. See text for further explanation
19.1.1.3.2.1 Barotrauma and Volutrauma
Historically, attention was focused on clinically apparent barotrauma, represented by air leak syndromes. Webb et al. were the first to demonstrate experimental evidence that high airway pressures could lead to increased capillary permeability, non-hydrostatic pulmonary edema, and tissue damage (Webb and Tierney 1974). Subsequent studies showed that ventilation with large tidal volumes had more impact on the occurrence of ventilator-induced lung injury than high airway pressures on itself (Carlton et al. 1990; Dreyfuss et al. 1992; Hernandez et al. 1989). Therefore, it has been advocated to replace the term “barotrauma” by “volutrauma” (Dreyfuss and Saumon 1992). The basic premise is that high tidal volumes (volutrauma) cause overdistension of alveoli, which is associated with increased capillary permeability, pulmonary edema, and histological damage (Carlton et al. 1990; Dreyfuss et al. 1985; Kim and Crandall 1982; Parker et al. 1984, 1990).
19.1.1.3.2.2 Atelectotrauma
Another putative mechanism causing ventilator-induced lung injury is the concept of “atelectotrauma.” Atelectotrauma is thought to be caused by repetitive opening and closing of alveoli resulting in shear stress and mechanical damage, especially in already diseased parts of the lung (Muscedere et al. 1994; Sohma et al. 1992; Taskar et al. 1997). In the previously mentioned study by Webb et al., it was already demonstrated that positive end-expiratory pressure as opposed to zero end-expiratory pressure had a protective effect (Webb and Tierney 1974).
19.1.1.3.2.3 Biotrauma
Finally, prolonged injurious ventilation results in microscopic abnormalities with inflammatory infiltrates that are indistinguishable from acute respiratory distress syndrome in humans (Kawano et al. 1987; Tsuno et al. 1991). Experimental studies showed a distinctive effect of ventilation strategy on cytokine concentrations in lung lavage (Tremblay et al. 1997). Harmful ventilation strategies were associated with major increases in cytokine concentrations. The term “biotrauma” has been coined to describe potentially injurious local and systematic inflammatory response to physiological stress.
Putting it all together, the sequence of events resulting in ventilator-induced lung injury can be described as depicted in Fig. 19.1.
19.1.1.3.3 Ventilation Strategies That Prevent Ventilator-Induced Lung Injury
Lung protective ventilation strategies specifically aim to prevent ventilator-induced lung injury. In both HFOV and CMV, these strategies rely on the same principles: recruitment and prevention of subsequent de-recruitment of alveoli while at the same time avoidance of overdistension. The concept of a lung protective ventilation strategy can be visualized by the pressure-volume curve. In this curve, a “safe window” seems to exist (see Fig. 13.1) (Froese 1997). This means that level of positive end-expiratory pressure must be sufficiently high to prevent de-recruitment and atelectasis on the one end and on the other end the peak inspiratory pressure superimposed on the positive end-expiratory pressure should not result in overdistension. The price of limiting tidal volumes to achieve this goal may be hypercapnia.
19.1.1.3.3.1 Prevention of Atelectasis
Prevention of atelectasis has been expressed as “open up the lung and keep the lung open” (Lachmann 1992). This implies that a sufficient amount of airway pressure is needed to maintain patency of the airways and alveoli. Positive end-expiratory pressure is pivotal in this concept and defines the lower limit of the pressure swings that can be applied in a lung protective ventilation strategy.
19.1.1.3.3.2 Prevention of Overdistension
Overdistension of alveoli has received attention first by limiting inspiratory pressures. More recently, emphasis has been put on limiting tidal volumes. Still, inspiratory pressures remain important proxies to estimate the level of overdistension. High positive pressure combined with limitation of inspiratory pressure results in even smaller tidal volumes to meet the demands of lung protective ventilation. In CMV, hypercapnia and respiratory acidosis will limit the feasibility to achieve the objectives of lung protective ventilation strategies.
19.1.1.3.3.3 Resulting Hypercapnia
The resulting hypercapnia, however, has been proposed to have an independent lung protective effect. It is unknown if hypercapnia can be considered as an acceptable side effect of a lung protective ventilation strategy or if it should be implemented in a lung protective ventilation strategy to strive for as an independent goal (Feihl and Perret 1994).
19.1.1.3.4 How Does HFOV Prevent Ventilation-Induced Lung Injury?
The lung protective ventilation strategy used in HFOV has been qualified as “high lung volume strategy.” A high lung volume strategy consists of the following approaches: (1) use of a higher mean airway pressure than on CMV, (2) initial weaning of fractional inspired oxygen before decreasing mean airway pressure, and (3) use of alveolar recruitment maneuvers. Fractional inspired oxygen is considered to be a useful clinical parameter to assess lung volume recruitment. Optimal recruitment is reflected by a fractional inspired oxygen which can be weaned below 0.30 or even 0.25.
19.1.1.3.4.1 Recruitment “Open Up the Lungs”
Recruitment of non-aerated lung tissue has become one of the three cornerstones of lung protective ventilation (Clark et al. 2000; Gattinoni et al. 2003). In applying an open-lung strategy, mean airway pressure is gradually increased by steps of 1–2 cm H2O, with the goal of reducing fractional inspired oxygen to less than 0.60–0.40. Oxygen saturation should remain above 90 % (or equal to 85 % in the premature infant). During this phase hemodynamics should be carefully monitored. Central venous pressure in pediatric patients should be maintained at a level sufficient to overcome mean airway pressure, typically around 10–15 mmHg (Arnold et al. 1994). In pediatric patients, additional inotropic support should be considered as well. In premature infants, at the occurrence of a minimal decrease of blood pressure (i.e., more than 2 mmHg), airway pressures should be reduced and the oxygen response conserved before eventually further increasing airway pressures again.
19.1.1.3.4.2 Optimizing Mean Airway Pressure “Keep the Lungs Open”
After recruitment, once appropriate saturation is achieved and fractional inspired oxygen can be lowered to less than or equal to 0.6, airway pressure should be reduced stepwise in decrements of 1–2 cm H2O (moving downward on the deflation limb of the pressure-volume curve that exhibits hysteresis) (Mehta et al. 2001; Rimensberger et al. 1999). As long as oxygen saturation can be maintained, airway pressure can be further decreased, until the lowest pressure is reached that ensures adequate saturation. Chest radiography is recommended after finding the optimal mean airway pressure to assess adequacy of lung recruitment and to exclude signs of overinflation. If signs of overinflation are present, airway pressures should be reduced even further.
19.1.1.3.4.3 Limiting Tidal Volumes “Prevent Overdistension”
In CMV, small tidal volume ventilation may cause complications resulting from the effects of acute respiratory acidosis on hemodynamics, gas exchange, and oxygen transport or consumption (Crotti et al. 2001) that require an increased use of sedatives and often muscle relaxants, and may lead to alveolar instability and lung collapse (Carvalho et al. 1997). Within the context of ventilator-induced lung injury and lung protective strategies, HFOV could be considered to be the most optimal protective ventilator mode, providing by design small tidal volume ventilation and facilitating lung recruitment and maintenance of optimal lung volume without concomitant lung overdistension (Ferguson and Slutsky 2008). However, there is ongoing debate whether this translates to better clinical outcome.
19.1.1.4 Elective vs. Rescue HFOV
Indications for HFOV can be divided in:
1.
Rescue indications: in which CMV is unable to maintain adequate gas exchange or has resulted in major complications
2.
Elective indications: in which HFOV is selected as the initial choice of treatment based on the expected improvement in clinical outcome as compared to CMV as an initial treatment choice
There is a tension between selecting HFOV as a rescue treatment and HFOV as a first-choice treatment. It has been argued that delayed use of HFOV in very low-birth-weight infants precluded the beneficial effects, as lung injury already might have occurred on CMV (Rimensberger et al. 2000). This has not been confirmed in meta-analysis of trials comparing HFOV with CMV in premature neonates (Bollen et al. 2007). In a meta-analysis of trials in adult patients with acute respiratory distress syndrome, duration on CMV prior to HFOV was not associated with outcome either (Bollen et al. 2006). However, in an individual patient data meta-analysis, the timing of initiation of HFOV has been suggested as a modifying factor of the relative treatment effect in a selected patient group of premature infants.
The ideal timing of HFOV, to make a difference with CMV, will be determined by the risk of ventilator-induced lung injury at a time lung injury has not occurred yet. At the extremes there are patients that are not at risk of ventilator-induced lung injury, and there are patients in whom poor pulmonary outcome cannot be avoided. HFOV will not exhibit any advantageous effects over CMV in these patients. Therefore, prognostic factors of ventilator-induced lung injury could serve as important indicators to initiate HFOV. It has been suggested that these markers relate to the inability of CMV to maintain tidal volumes within the safe window of the pressure-volume curve while preserving adequate gas exchange (Bollen et al. 2008).
19.1.1.5 Choosing the Right Patient for HFOV
19.1.1.5.1 Is Recruitment Possible?
Adult and newborn animal studies have shown that the full potential of attenuating ventilator-induced lung injury will only be reached if HFOV is combined with optimal recruitment and stabilization of previously atelectatic lung units (Kolton et al. 1982; McCulloch et al. 1988; Meredith et al. 1989). One of the major “handicaps” in optimizing recruitment or lung volume during HFOV in clinical practice is the inability to accurately measure direct changes in lung volume at the bedside (Hulskamp et al. 2006). Animal studies suggested that oxygenation can be used as an indirect measure of change in lung volume (Suzuki et al. 1992; van Genderingen et al. 2004). The relative benefit of HFOV over CMV could be determined by the extent to which optimization of recruitment and change of lung volume, i.e., oxygenation, is feasible in the individual patient. In patients with lungs that cannot be recruited, so-called baby lungs (Gattinoni et al. 2004), HFOV could prevent overdistension while providing adequate ventilation as compared to CMV.
19.1.1.5.2 The Importance of Ventilation Strategies
Specific lung protective ventilation strategies utilized in CMV can reduce chronic lung disease. Treatment strategies and their effects on lung injury are based on pathophysiological studies in animal models, showing increased cytokine release with higher tidal volumes and reduced positive end-expiratory pressure (Meredith et al. 1989), or trials in adults with respiratory distress syndrome (Petrucci and Iacovelli 2007). Allowing carbon dioxide to rise, permissive hypercapnia, rather than increasing ventilation reduces lung injury in preterm infants (Woodgate and Davies 2001). Strategies to synchronize ventilation using higher respiratory rates and patient-triggered ventilation also reduce the rate of pneumothorax and the duration of ventilation (Greenough et al. 2008).
Clark et al. pointed out the importance of ventilation strategies employed in HFOV and CMV to compare the relative treatment effect of reducing lung injury (Clark et al. 2000). The importance of ventilation strategies, as such, compared to the type of ventilator that delivers these strategies has been demonstrated in a cumulative meta-analysis of trials in premature infants (Bollen et al. 2003). In this study, the modifying effects on the relative treatment benefit of HFOV over CMV of ventilation strategies and concomitant treatment were shown. Enhancements of CMV over the years have diminished the relative treatment benefits of HFOV.
19.1.1.5.3 Classical Indications for HFOV in Neonatal and Pediatric Patients
Classical indications for HFOV in the neonatal population are as follows: respiratory distress syndrome, congenital diaphragmatic hernia, air leak syndrome, and persistent pulmonary hypertension of the newborn (Bryan and Cox 1999; Nobuhara and Wilson 1996). Classical indications for HFOV in the pediatric population include diffuse alveolar disease (e.g., primary or secondary acute respiratory distress syndrome) (Arnold et al. 1994), with growing experiences of lower airway disease, at least when conventional ventilation fails (bronchiolitis, status asthmaticus, and acute chest syndrome) (Berner et al. 2008; Wratney et al. 2004; Duval and van Vught 2000; Duval et al. 2000).
19.1.1.5.4 Indications in Adult Patients
Evidence obtained in adult patients can be of considerable relevance to larger pediatric patients. HFOV has been used in adults for a variety of indications. The most important indication for HFOV is acute respiratory distress syndrome as in pediatric patients (Mehta et al. 2001; Derdak et al. 2002; Fort et al. 1997). Chan et al. summarized the evidence of use of HFOV in adult patients with acute respiratory distress syndrome (Chan et al. 2007). They concluded that many questions remained unanswered and, given the substantial mortality reductions with current CMV based lung protective strategies, HFOV should be compared to CMV in a large randomized trial with mortality as the primary outcome measure.
Table 19.1 summarized the different indications for HFOV in specific patient groups along with the levels of evidence that support these indications.
Table 19.1
Levels of evidence to use HFOV instead of CMV
Indication |
Patients |
Type of use |
Clinical effect |
Level of evidence |
References |
---|---|---|---|---|---|
Respiratory distress syndrome |
Preterm neonates |
Elective |
Reduction of chronic lung disease, estimated number to treat = 25 |
I-A |
|
Long-term outcome is similar with respect to pulmonary function and neurodevelopmental outcome |
I-B |
||||
Rescue |
There is insufficient evidence to estimate the clinical effect of rescue HFOV in preterm neonates |
I-B |
|||
Term or near-term neonates |
Rescue |
Successful weaning from mechanical ventilation with HFOV after failure of HFOV |
III |
Ben et al. (2006a) | |
Pulmonary dysfunction |
Term or near-term neonates |
Rescue |
No clinical benefit with or differences in treatment failure between HFOV and CMV |
I-A |
|
Diaphragmatic hernia |
Neonates |
Elective |
Improvement of survival has been recorded in selected patients |
III |
|
Severe pulmonary hypertension |
Neonates |
Rescue |
HFOV combined with inhaled nitric oxide could prevent ECMO. However, benefit over CMV with inhaled nitric oxide has not been demonstrated |
I-A |
Kinsella et al. (1997a) |
Acute respiratory distress syndrome |
Pediatric patients |
Rescue |
Improvement in oxygenation and reduced need for oxygen at 30 days, but no reduction in mortality |
I-B |
Arnold et al. (1994) |
Adult and pediatric patients |
Rescue |
No significant reduction in mortality. However, there is improvement in short-term oxygenation and there is a trend toward reduced mortality |
I-A |
||
Small airway disease |
Pediatric patients |
Rescue |
Achieving adequate gas exchange in selected patients when CMV fails |
III |
|
Asymmetric lung injury |
Pediatric and adult patients |
Rescue |
HFOV is feasible in asymmetric lung injury. However, clinical benefit is unknown |
III |
|
Air leak syndromes |
Pediatric and adult patients |
Rescue |
HFOV in these patients can have mixed results. Only in selected patients apparent clinical benefit compared to CMV has been shown |
III |
|
Acute lung injury following trauma |
Adult patients |
Rescue |
Clinical benefit seems to be comparable with other patients with acute respiratory distress syndrome |
III |
|
Burn and smoke inhalation |
Pediatric and adult patients |
Rescue |
HFOV seems beneficial in patients with only burn but no inhalation injury |
III |
|
Acute respiratory distress with brain trauma |
Pediatric and adult patients |
Rescue |
Raised intracranial pressure does not present an absolute contraindication in selected patients with concomitant lung injury |
III |
|
Acute chest syndrome |
Pediatric patients |
Rescue |
Refractory hypoxemia on CMV can be improved with HFOV |
III |
Wratney et al. (2004) |
19.1.1.6 HFOV in the Neonatal Intensive Care Unit
19.1.1.6.1 Elective HFOV in Respiratory Distress Syndrome
19.1.1.6.1.1 Short-Term Outcome
Pulmonary disease continues to be a major cause of morbidity and mortality in very preterm infants. Although CMV has decreased mortality, morbidity from lung injury remains high. Acute injury such as pulmonary air leak was common prior to the availability of surfactant. However, chronic lung disease still develops in up to one third of preterm infants with respiratory distress syndrome who require mechanical ventilation (Lemons et al. 2001; Northway 1992). In addition to immaturity, overdistension of the lung and oxygen toxicity are considered to be important causal factors in the pathogenesis of chronic lung disease (Jobe and Ikegami 2000).
The focus in very preterm patients has been on the elective use of HFOV ventilation. Premature neonates most at risk for chronic lung disease have been included in studies comparing HFOV and CMV as first choice. Although data suggest significant benefits in pulmonary outcomes when HFOV is applied with a recruitment strategy in preterm infants with respiratory distress syndrome, when compared with CMV, it is still not clear whether HFOV is really superior in terms of overall outcome in this population, as careful CMV may allow investigators to achieve similar outcome data (Bollen et al. 2003). Two large multicenter trials that emphasized alveolar recruitment as part of the strategy in HFOV dominate current evidence (Courtney et al. 2002; Johnson et al. 2002). The US trial used a strategy in the CMV group that targeted a tidal volume of 5–6 mL/kg of body weight. This study showed a statistically nonsignificant reduction in days on the ventilator (13 days in the HFOV group vs. 21 days in the CMV group) and a significant decrease in the need for supplemental oxygen at 36 weeks of gestational age in favor of HFOV (Courtney et al. 2002). The other trial, the UK Oscillation Study, utilizing not as vigorously controlling ventilator protocols, could show no difference in outcome between the two study arms (Johnson et al. 2002).
Low gestational age or birth weight, intrauterine growth restriction, severity of lung disease, and exposure to perinatal inflammation are considered important risk factors (Egreteau et al. 2001). Differences in these risk factors result in different risk profiles for chronic lung disease. This could explain inconsistency in occurrence of chronic lung disease between trials, as inclusion of infants with a very low or a very high a priori risk for chronic lung disease may override any treatment effect of the ventilation strategy if not compensated by an adequate sample size (van Kaam and Rimensberger 2007).
19.1.1.6.1.2 Long-Term Outcome
Neuromotor outcome has been of concern in HFOV, as one of the first trials reported increased risk of intraventricular hemorrhage and periventricular leukomalacia associated with HFOV (The HIFI Study Group 1989). However, this trial was criticized because the methodology used to apply HFOV did not include methods to recruit lung volume (Bryan and Froese 1991). Moreover, in a trial which initially demonstrated a higher incidence of intraventricular hemorrhage in HFOV-treated neonates, at 2 years of follow-up, neuromotor outcome was comparable to CMV (Truffert et al. 2007). Furthermore, neurodevelopmental outcome was studied in children that entered into the UK Oscillation Study, which was designed to evaluate these outcomes (Johnson et al. 2002). The prevalence of severe neurodevelopmental disability was similar in children who received HFOV or CMV (relative risk 0.93, 95 % confidence interval 0.74–1.16) (Marlow et al. 2006). Long-term pulmonary function, frequency of hospitalization, or disabilities were not found to differ among children treated with HFOV or CMV either (Gerstmann et al. 2001; Thomas et al. 2004).
19.1.1.6.1.3 Recommendation
HFOV as a first-line therapy of respiratory distress in premature neonates does not offer convincing benefits compared to CMV to be accepted as a sole treatment option for these patients. Application of ventilation strategy is likely to be more important than type of ventilator. However, carefully applied CMV can only yield comparable results with HFOV as long as the targets of lung protective ventilation can be met. Failure to recruit the lungs, to limit tidal volumes, or to accept hypercapnia can prompt for the use of HFOV as an alternative therapy. On the other hand, carbon dioxide levels and hemodynamic parameters have to be carefully monitored, and a high lung volume strategy should be employed when using HFOV, to avoid neurological damage.
19.1.1.6.2 Rescue HFOV in Respiratory Distress Syndrome
Timing of initiating of HFOV has been considered to be an important factor. Animal experiments have demonstrated that only a few breaths during CMV could suffice to trigger the cascade of ventilator-induced lung injury (Bjorklund et al. 1997). Delayed start of HFOV could, therefore, attenuate potency for beneficial effects (van Kaam and Rimensberger 2007). Rescue use of HFOV in preterm infants is only described in one trial. This trial was performed in the pre-surfactant era, limiting interpretation of results (HiFO Study Group 1993; Bhuta and Henderson-Smart 2000). Early rescue use of HFOV has been studied in nearly term and term infants. Neonates with gestational age >34 weeks were managed with HFOV if they required fractional inspired oxygen above 0.5 with mean airway pressure above 10 cm H2O. Seventy-seven children fulfilled these criteria of which 70 (91 %) could be successfully weaned from HFOV (Ben et al. 2006a). A Cochrane review of the rescue use of HFOV in newborns more than 34 weeks gestation found only one randomized controlled study. This rescue trial of 79 infants (58 % met ECMO criteria), conducted by Clark et al., showed improvement in gas exchange in 63 % of the patients in whom CMV failed, whereas use of CMV, after failure of HFOV, was not effective (Clark et al. 1994). There were no significant differences in the numbers of patients requiring ECMO, days on a ventilator, days on oxygen, or days in the hospital between the two groups.
19.1.1.6.2.1 Recommendation
Rescue use of HFOV after CMV failure is indicated to improve short-term improvements of gas exchange. However, there is no convincing evidence to recommend HFOV to improve long-term outcome. It could be argued that once CMV has failed, prognosis cannot be enhanced by HFOV and, therefore, HFOV should be initiated earlier.
19.1.1.6.3 HFOV for Other Indications
Randomized trials that studied HFOV in neonates included mostly patients with respiratory distress syndrome. The efficacy of HFOV to attenuate ventilator-induced lung injury in other causes of respiratory failure, therefore, for the larger part remains to be investigated.
19.1.1.6.3.1 Severe Pulmonary Dysfunction and Intractable Respiratory Failure
Two trials have studied the use of HFOV compared to CMV in term or near-term infants with severe pulmonary dysfunction (Henderson-Smart et al. 2009). One trial, involving “elective” use of HFOV, randomized 118 infants at the start of CMV (Rojas et al. 2005). The other trial of “rescue” HFOV randomized 81 infants with respiratory failure already on CMV (Clark et al. 1994). Neither trial showed evidence of a reduction in mortality at 28 days or in failed therapy on the assigned mode of ventilation requiring crossover to the other mode. Neither study reported significant differences in the risk of pulmonary air leak, chronic lung disease (28 days or more on oxygen), or intracranial injury.
19.1.1.6.3.2 Recommendation
There is no convincing evidence to support the use of HFOV in neonates with severe pulmonary dysfunction or intractable respiratory failure. However, evidence is scarce and more research is needed to identify patients that might benefit from HFOV.
19.1.1.6.3.3 Diaphragmatic Hernia
Several descriptive studies report outcome of HFOV in neonates with diaphragmatic hernia (Datin-Dorriere et al. 2008; Migliazza et al. 2007). In a retrospective study neonatal outcome of isolated congenital diaphragmatic hernia treated with early HFOV and delayed surgery was analyzed. Survival rate at 1 month was 65.9 % (Datin-Dorriere et al. 2008). Migliazza et al. described a similar experience adopting a “gentle ventilation” strategy, using HFOV, of 111 infants with congenital diaphragmatic hernia (Migliazza et al. 2007). The overall survival rate was 69.4 %, comparable to Datin-Dorriere et al.
19.1.1.6.3.4 Recommendation
Evidence of HFOV in diaphragmatic hernia is restricted to descriptive studies. This establishes HFOV as a feasible treatment option but not as the recommended therapy in this group of patients.
19.1.1.6.3.5 Severe Persistent Pulmonary Hypertension
In a randomized multicenter trial, the use of HFOV combined with nitric oxide in severe persistent pulmonary hypertension of the newborn has been investigated (Kinsella et al. 1997a). In this study, the combination of HFOV with nitric oxide was more beneficial than nitric oxide with CMV or HFOV alone. However, treatment success was defined as response rate and differences in clinical relevant outcomes, or prevention of extracorporeal membrane oxygenation could not be assessed by design of the trial.
19.1.1.6.3.6 Recommendation
Although HFOV seems a viable treatment option for treatment failure of CMV combined with inhaled nitric oxide, there is no definitive evidence that HFOV is more effective in preventing extracorporeal membrane oxygenation or improving clinical outcome than CMV combined with inhaled nitric oxide.
19.1.1.7 HFOV in the Pediatric Intensive Care Unit
In pediatric intensive care unit patients, there is less evidence to direct selection of HFOV. Evidence has to be translated from studies performed in neonatal or adult patients. In only one randomized trial HFOV has been compared to CMV in pediatric patients with acute respiratory distress syndrome (Arnold et al. 1994). No randomized trials have been performed that looked at the elective use of HFOV compared to CMV. Yet, use of HFOV has become ingrained in pediatric practice and is used frequently in children with acute respiratory distress syndrome, despite the lack of evidence to support this (Randolph et al. 2003).
19.1.1.7.1 HFOV in Acute Respiratory Distress Syndrome
The first description of acute respiratory distress syndrome originates from Ashbaugh describing 12 patients with acute respiratory distress, cyanosis refractory to oxygen therapy, decreased lung compliance, and diffuse infiltrates evident on chest X-ray (Ashbaugh et al. 1967). According to a prospective cohort study, the prevalence of acute respiratory distress syndrome or acute lung injury is about 9 % among adult intensive care patients and 39.6 % among ventilated patients (Roupie et al. 1999). In pediatric patients, acute respiratory distress is a more rare syndrome with incidences ranging from 1.4 to 7.9 % (Yu et al. 2009; Dahlem et al. 2003). Mortality, however, is high ranging from 31.4 to 61 %, particularly when compared to normal PICU mortality. A hallmark feature of acute respiratory distress syndrome is low compliance, necessitating high pressures to maintain sufficient tidal volume. Tidal volume, which is large relative to lung compliance, may lead to alveolar rupture and radiological evidence of extra-alveolar air. Distribution of tidal volume to the high-compliance regions causes stretching and sheer forces on the alveolar wall (Dreyfuss and Saumon 1998; Parker et al. 1993). When parts of the lung are consolidated, e.g., not recruitable, a “normal” tidal volume may still lead to overstretching of remaining alveoli, increasing already existent lung disease in acute respiratory distress syndrome.
19.1.1.7.1.1 HFOV for Acute Respiratory Distress Syndrome in Pediatric Patients
One crossover trial comparing rescue HFOV with CMV in pediatric acute lung injury and acute respiratory distress syndrome showed that HFOV was associated with higher mean airway pressures, improved oxygenation, and a reduced need for supplemental oxygen at 30 days (Arnold et al. 1994). In a prospective clinical study, HFOV in 20 pediatric patients with acute respiratory failure due to a variety of causes showed improvement of gas exchange in a rapid and sustained fashion (Ben et al. 2006b).
19.1.1.7.1.2 HFOV for Acute Respiratory Distress Syndrome in Adult Patients
The use of HFOV in the context of acute respiratory distress syndrome in adult patients has been studied in 4 randomized trials (Derdak et al. 2002; Bollen et al. 2005; Young et al. 2013; Ferguson et al. 2013). In the first trial of 148 patients there was a nonsignificant lower 30-day mortality in the HFOV group (37 % vs. 54 %, p = 0.10). However, the CMV protocol did not use the current standard for volume- and pressure-limited lung protective ventilation, and the study was not powered to detect mortality differences since mortality was only a secondary outcome criteria (Derdak et al. 2002). A second trial of 61 acute respiratory distress syndrome patients comparing HFOV with CMV also revealed no significant differences in survival without supplemental oxygen or ventilation support (32 % vs. 38 %, adjusted odds ratio 0.80, 95 % confidence interval 0.22–2.97, p = .79) (Bollen et al. 2005). A post hoc analysis suggested that patients with the most severe hypoxemia had a trend toward a benefit from HFOV. In contrast, recently two large RCTs comparing HFOV with a conventional lung protective ventilation, the Oscillation in ARDS (OSCAR) (Young et al. 2013) and the Oscillation for Acute Respiratory Distress Syndrome Treated Early (OSCILLATE) (Ferguson et al. 2013) trials, showed either no benefit (for the OSCAR trial) or even harm (for the OSCILLATE trial) with HFOV in adults with early moderate-to severe ARDS. The OSCILLATE trial has been stopped after 548 patients (planned 1200) on the recommendation of the data monitoring committee because of an unexpected high in-hospital mortality of 47% in the HFOV group, as compared with 35% in the control group (relative risk of death with HFOV, 1.33; 95% confidence interval, 1.09 to 1.64; p = .005). In this trial the patients randomized to HFOV received more sedation (p < .001), neuromuscular blockers (p < .001), and vasoactive drugs (p = .01) than those randomized to CMV. This latter suggests that the recruitment protocol and the high volume strategy with reported mean airway pressures of more than 30 cmH2O during HFOV after recruitment might have caused lung overdistention and hemodynamic compromise, which might explain the high 30-day mortality. For this reason this trial has been criticized repeatedly (Malhorta and Drazen 2013; Liaudet 2013; Guervilly et al. 2013).
19.1.1.7.1.3 Acute Respiratory Distress Syndrome, Timing of HFOV
The use of HFOV is considered to be a safe alternative to CMV in adult patients with severe acute respiratory distress syndrome who have failed CMV (Chan and Stewart 2005). Immediate use of high-frequency ventilation in patients with acute respiratory distress syndrome has been advocated (Goutorbe et al. 2008). However, further research is needed to determine the ideal patients, timing, and optimal technique with which to provide HFOV.
Oxygenation failure has been classically an indication for HFOV. Oxygenation failure requires a ventilation strategy in which positive end-expiratory pressure is raised to limit fractional inspired oxygen while at the same time peak inspiratory pressures and tidal volumes have to be limited as well. Table 19.2 summarizes the criteria reported in literature to initiate HFOV in oxygenation failure.
Table 19.2
Recommendations to initiate HFOV in acute respiratory distress syndrome
Indications |
References | |
---|---|---|
1 |
An apparent oxygenation failure using CMV, i.e., the need for an FiO2 >0.60, despite optimizing PEEP, inverse ratio ventilation, or other conservative measures to improve oxygenation such as adequate circulatory support |
|
High inspiratory pressures needed to maintain adequate oxygenation or resulting tidal ventilation volumes in CMV that are too small to result in sufficient carbon dioxide elimination, resulting in respiratory acidosis (i.e., pH < 7.20–7.25) | ||
2 |
Oxygenation failure: FiO2 ≥0.7 and positive end-expiratory pressure >14 cm H2O |
Fessler et al. (2007) |
Ventilation failure: pH < 7.25 with tidal volume ≥6 mL/kg predicted body weight and plateau airway pressure ≥30 cm H2O | ||
3 |
FiO2 >0.60 and SpO2 <88 % on conventional ventilation with positive end-expiratory pressure >15 cm H2O |
Higgins et al. (2005) |
Plateau pressures >30 cm H2O | ||
Mean airway pressure >24 cm H2O | ||
Airway pressure release ventilation high pressure 35 cm H2O |
The oxygenation index has been proposed as a candidate marker to determine the timing of transition of CMV to HFOV in case of oxygenation failure (Bollen et al. 2005; van Genderingen et al. 2002). The oxygenation index combines the most important characteristics of oxygenation failure and can be regarded as a cost-benefit indicator. The cost being fractional inspired oxygen and mean airway pressure and the arterial oxygen content as a measure of the benefit obtained. An oxygenation index above 20 could serve as a marking point to initiate HFOV in acute respiratory distress syndrome or intractable oxygenation failure.
19.1.1.7.1.4 Recommendation
There is no evidence that HFOV offers a clear clinical benefit over CMV (Wunsch and Mapstone 2004). However, in selected patients, HFOV could be indicated. Proposals for indications are listed in Table 19.2, but evidence to back up these protocols is still missing. Further research is needed to establish the position of HFOV as a second-line treatment in acute respiratory distress syndrome.
19.1.1.7.2 HFOV for Other Indications
Evidence of indications for HFOV other than acute respiratory distress syndrome is largely anecdotal. In these patient groups no randomized or controlled trials have been performed.
19.1.1.7.2.1 Small Airway Disease
In small airway disease adequate carbon dioxide removal is of prime concern. Carbon dioxide elimination is directly correlated with expiratory minute volume in CMV. HFOV, however, does not directly rely on tidal volume movement, but can be very efficient in carbon dioxide removal through alternative mechanisms. Airway obstruction and plugging in HFOV on the other hand can severely hamper carbon dioxide elimination. Use of HFOV in small airway disease has been suggested in patients in which higher mean airway pressure can stent the airways, preventing them from collapsing (Duval and van Vught 2000; Duval et al. 2000). The continuous distending airway pressure of HFOV can be used to open up and stent the small airways (“open-airway” concept in analogy to the “open-lung” concept).
HFOV has been used to treat respiratory failure due to RSV bronchiolitis (Berner et al. 2008). The proportion of infants with RSV infection requiring CMV is reported to vary around 25 % of those infants that are admitted to the intensive care unit (Larrar et al. 2006; Campion et al. 2006). Adequate mechanical ventilation in obstructive lung disease represents a challenge. High peak inspiratory pressures are needed to guarantee sufficient tidal volumes and minute ventilation (Leclerc et al. 2001). The use of HFOV has been described as rescue intervention in several cases to improve severe hypoxemic respiratory failure while carbon dioxide elimination was compromised (Kneyber et al. 2005; Thompson et al. 1995; Medbo et al. 1997; Duval et al. 1999). These reports show that respiratory failure with hypercapnia can be managed with HFOV using high mean airway pressure and large pressure swings while preserving spontaneous breathing. Use of HFOV in an infant with cystic fibrosis and viral bronchiolitis has been described as well. Severe hypercarbic respiratory failure was treated successfully with HFOV in this patient (Seferian et al. 2006).
The “open-airway” concept as an approach to ventilate patients with small airway disease is further described by Slee-Wijffels et al. in 17 patients along with 32 patients with diffuse alveolar disease (Slee-Wijffels et al. 2005). The latter group was characterized by oxygenation failure and a considerably higher mortality compared to patients with small airway disease. Using the “open-airway” concept HFOV was effective in achieving adequate ventilation in all of these patients.
Management of status asthmaticus has been described in a case report (Duval and van Vught 2000). It was suggested that obstructive airway disease could be safely managed, providing certain conditions are met: application of sufficiently high mean airway pressures to open and stent the airways (“an open-airway strategy”), lower frequencies to overcome the greater attenuation of the oscillatory waves, permissive hypercapnia, and longer expiratory times and muscle paralysis to avoid spontaneous breathing.
19.1.1.7.2.2 Recommendation
The use of HFOV in pulmonary conditions with increased airway resistance and prolonged time constants, such as small airway disease, remains controversial. Use of HFOV in these patient groups is anecdotal. Therefore, treatment of small airway disease with HFOV should be restricted to centers with extensive experience with HFOV and to those patients in whom conventional treatment options fail.
19.1.1.7.2.3 Asymmetric Lung Injury
HFOV has been used to selectively ventilate the collapsed lung in patients with asymmetric acute lung injury, while the more homogeneously infiltrated lung was managed by conventional ventilation (Terragni et al. 2005). The same strategy in asymmetric pulmonary disease has been described by Plotz et al. in a 14-year-old patient (Plotz et al. 2003). The first report on this particular use of HFOV was published by Pizov in the management of traumatic tear of bronchus in a child (Pizov et al. 1987). The rationale behind this treatment is that HFOV can thus be used to selectively apply a high mean airway pressure to the consolidated lung without creating alveolar overdistension and volutrauma in the relatively disease-free opposite lung.
19.1.1.7.2.4 Recommendation
This usage of HFOV should be considered experimental and should only be reserved for the very special cases in centers with experience in HFOV.
19.1.1.7.2.5 Air Leak Syndromes
HFOV in air leak syndromes can have mixed results. On the one hand, HFOV does not necessitate pressure swings as experienced in CMV. This could have a beneficial effect on the causative lesions of the air leak syndrome. On the other hand, higher mean airway pressure in HFOV can be hypothesized to maintain the air leak. Only case reports of the use of HFOV specifically in air leak syndromes have been published (Galvin et al. 2004). HFOV has been used in traumatic tracheal laceration in a pediatric patient (Markus-Rodden et al. 2008). HFOV was initiated in this case because of concomitant lung injury resulting in deteriorating respiratory status. Another case report describes the successful use of HFOV in a high output bronchopleural fistula (Ha and Johnson 2004). A case of Lemierre disease in a 14-year-old girl resulting in cavitating pneumonia was managed by a combined therapy of nitric oxide and HFOV (Briggs et al. 2003). A case report describes the use of HFOV to manage pneumatoceles. It was argued that CMV could lead to distension of the pneumatoceles through air-valve mechanisms (Shen et al. 2002). HFOV using a permissive hypercapnia and hypoxemia strategy has also been used in pediatric acute respiratory distress syndrome with pneumomediastinum (McGinley et al. 2001).
19.1.1.7.2.6 Recommendation
HFOV cannot be recommended as a first-line therapy as only anecdotal reports describe its use in these patients. If HFOV is used, fractional inspired oxygen should be increased in favor of airway pressure.
19.1.1.7.2.7 Acute Lung Injury Following Trauma
Use of HFOV has been studied as a rescue therapy for adult trauma patients (Briggs et al. 2009). This retrospective study of 24 patients concluded that although HFOV improved oxygenation, severity of traumatic injury and organ failure were predictors of survival, not respiratory status. In trauma patients with severe pulmonary contusion, HFOV has been used as rescue therapy (Funk et al. 2008). Rapid improvement in oxygenation index and PaO2/FiO2 ratio was achieved. HFOV in surgical patients appeared to be safe and effective in correcting oxygenation failure in 16 patients that failed on CMV (Kao et al. 2006). A considerable increase in PaO2/FiO2 ratio was observed that was maintained throughout the study after initiation of HFOV.
19.1.1.7.2.8 Recommendation
Not surprisingly, HFOV to treat lung injury after trauma shows comparable results to HFOV in treatment of acute respiratory distress syndrome. However, evidence in these patients is anecdotal and does not seem to represent a group different from other patients with acute lung injury or acute respiratory distress syndrome.
19.1.1.7.2.9 Burn and Smoke Inhalation Patients
The associated risk of mortality in acute lung injury due to smoke inhalation has been reported as high as 40–50 %. Mortality may be due directly to respiratory failure and inability to oxygenate sufficiently, or it may result from associated multisystem organ failure or ventilator-associated pneumonia. Accepting hypercapnia when using low tidal volumes to prevent ventilator-induced lung injury, permissive hypercapnia, has been shown to be safe to a modest degree in these patients (Hickling et al. 1994; Laffey et al. 2004; Bidani et al. 1994). However, very little has been reported on the use of HFOV after inhalation injury, aside from one animal experiment (Cioffi et al. 1993), a pediatric case report (Jackson et al. 2002), and data from 19 adult burn and inhalation injury patients treated with HFOV (Cartotto et al. 2009; Cartotto 2009). The unique clinical and pathophysiologic features of smoke inhalation could pose limitations to the application of HFOV. Acute respiratory distress syndrome in smoke inhalation injury could be a more heterogeneous disease resulting in non-recruitable alveoli thereby limiting the beneficial effects of high-frequency ventilation by opening and recruiting alveoli (Crotti et al. 2001; Gattinoni et al. 2006). Patients with burns and smoke inhalation were found to respond less vigorously to HFOV as those with burn injury alone. Those with inhalation injury failed to achieve significant improvement in PaO2/FiO2 ratio or oxygenation index compared with baseline on CMV before HFOV and never obtained a significant reduction in oxygenation index (Cartotto et al. 2009). This contrasted sharply with non-inhalation cases where there was a significant improvement in oxygenation within 8 h of HFOV.
19.1.1.7.2.10 Recommendation
Based on limited evidence, HFOV cannot be recommended to treat isolated inhalation injury. Only in burn patients with acute respiratory distress syndrome, HFOV could have beneficial effects.
19.1.1.7.2.11 Acute Lung Injury with Concurrent Brain Injury
Modern management of acute traumatic brain injury in children aims to minimize secondary physiologic insults such as raised intracranial pressure, reduced cerebral perfusion pressure, and hypotension or hypoxemia by optimizing cerebral perfusion and oxygenation while the brain recovers (Jones et al. 2003). CMV is used routinely in these patients to prevent hypercapnia and hypoxia during the acute phase. Effective gas exchange, however, can be problematic in those with coexisting lung pathology. Lung protective ventilation strategies used in CMV could conflict with therapeutic goals in treatment of traumatic brain injury. HFOV in these children has raised concerns about the potential adverse effects on the cerebral venous drainage and intracranial pressure. In a case report of two head-injured children, HFOV was used to handle concomitant traumatic lung injury. In both these patients, a dramatic reduction in intracranial pressure was observed soon after the use of HFOV (Lo et al. 2008). A comparable case series of five patients reached the same conclusion that HFOV appeared to be a relatively safe and effective means of oxygenating patients with severe acute respiratory distress syndrome and concomitant increased intracranial pressure due to acute brain injury (Bennett et al. 2007). HFOV appeared a safe rescue treatment in head-injured patients in a case series of five adult patients with severe respiratory insufficiency as well (David et al. 2005).
19.1.1.7.2.12 Recommendation
Raised intracranial pressure, classically considered a contraindication for HFOV, does not seem an absolute contraindication in patients with concomitant respiratory failure. In these patients, when intracranial pressure is monitored, HFOV can be an alternative to CMV.
19.1.1.7.2.13 Acute Chest Syndrome
Severe acute chest syndrome can cause hypoxemia refractory to conventional treatment. Obstructive mucus plugging and the development of acute respiratory distress syndrome may underlie the pathophysiology of refractory hypoxemia in acute chest syndrome. In disease processes with high airway resistance and obstructive mucus plugging, HFOV may predispose to air trapping and increased morbidity secondary to air leak syndromes. A case series of six pediatric patients describe the successful treatment of acute chest syndrome found in sickle cell anemia (Wratney et al. 2004). Patients failing CMV could be safely managed by HFOV, without strong concern about impaired secretion clearance or air leak syndromes.
Future Perspectives
-
In neonatal patients, the tendency is strongly in favor of avoiding invasive ventilation. Once invasive ventilation is inevitable, baseline risk of chronic lung disease could determine whether or not HFOV could have clinical benefits over CMV. Heterogeneity among trials that studied the elective use of HFOV could be explained by differences in baseline risk of chronic lung disease. However, CMV with careful lung protective ventilation strategy seems to yield comparable results.
-
In pediatric patients it is not to be expected that HFOV will replace CMV as the first-choice mode of mechanical ventilation. Indications for HFOV transpire when lung protective ventilation strategies are no longer feasible using CMV. Indications for HFOV could depend more on respiratory-dependent predictors of ventilator-induced lung injury than on specific disorders, like acute respiratory distress syndrome. High fractional oxygen requirement combined with raise mean airway pressures as reflected by the oxygenation index could be used to identify patients most at risk of ventilator-induced lung injury and thus could indicate when to apply HFOV.
-
Individual patients with varying lung pathology and heterogeneous disease patterns will require individual assessment of which ventilation settings will be most optimal. Conditional on those factors it should be determined whether or not HFOV offers benefits over CMV. Unfortunately, convincing evidence to guide these decisions is still missing.
19.1.1.7.2.14 Recommendation
Acute chest syndrome can be managed by HFOV. However, the evidence is anecdotal and only shows the feasibility of using HFOV in these patients.
Essentials to Remember
-
There are no strict, absolute indications to use HFOV in favor of CMV. Moreover, the use of HFOV should not be regarded as the key to improve outcome but as a means to enable lung protective ventilation. Only when lung protective ventilation is not feasible using CMV, HFOV could outperform CMV. Whether or not lung protective ventilation is possible and how this will change the prognosis with respect to ventilator-induced lung injury is determined by patient and disease characteristics.
-
The general aim of lung protective ventilation is to avoid overdistension on the one hand and atelectasis on the other hand. This can be visualized as a “safe window” on the pressure-volume curve. The size of this safe window can be translated to tidal volume. Thus, the smaller the safe window, the smaller the tidal volumes should be. In this respect, HFOV provides the theoretically most optimal ventilation mode. However, at present there are no conclusive studies that identify those specific patients.
-
The relatively high distending airway pressure, possible with HFOV, has been taken advantage of to “stent” the airway in small airway disease. However, evidence of the effectiveness for this indication is anecdotal, as is the evidence for use in air leak syndromes that uses HFOV with a relatively low mean airway pressure. In the latter it is thought that the absence of large pressure swings, in CMV, prevents maintaining air leak.
19.1.2 Indications for High-Frequency Jet Ventilation (HFJV)
Martin Keszler9
(9)
Division of Neonatology, The Children’s Hospital of Philadelphia, University of Pennsylvania, Philadelphia, PA, USA
Educational Aims
-
Describe the experimental evidence in support of specific clinical applications of HFJV.
-
Describe the clinical evidence in support of specific clinical applications of HFJV.
-
Indicate the limitations of available studies and their applicability to today’s patient population.
During the 1980s and 1990s, HFJV became firmly established as an important therapeutic alternative, based primarily on its effectiveness in treating air leak. However, despite more than 20 years of laboratory and clinical research with dozens of peer-reviewed publications, the exact role of HFJV (and other high-frequency ventilation techniques) remains controversial with no clear consensus emerging. At one end of the spectrum, a minority of clinicians use HFJV as a first-line mode of ventilation for extremely low-birth-weight infants they believe to be at high risk of chronic lung disease, while at the other extreme are those who view it strictly as a rescue technique, to be used only when conventional ventilation has clearly failed. Majority of clinicians appear to have a more measured approach, utilizing HFJV as an early rescue mode in infants who are judged to be at high risk of complications with conventional ventilation because they require high inspiratory pressure or have developed air leak complications, even though they are still maintaining adequate gas exchange on conventional ventilation.
The ongoing controversy regarding indications for HFV in general and HFJV in particular is related to the conflicting results of published clinical trials and the recognition that therapeutic options such as surfactant replacement therapy and synchronized, volume-targeted ventilation, which were not available when the early studies of HFV were conducted, limit the applicability of the conclusions to today’s neonatal patients. However, several well-supported indications do exist. In the following paragraphs I will briefly review available literature on HFJV in various disease processes and formulate evidence-based recommendations for the use of HFJV (Table 19.3).
Table 19.3
Proposed indications for HFJV
1 |
Air leak syndromes |
Level 1 evidence for PIE |
Level 3 evidence for BPF and TEF | ||
2 |
Severe uniform disease (RDS) |
Level 3 evidence (cohort and historical control studies) |
Rescue therapy | ||
3 |
Severe uniform disease (RDS) |
Level 1 evidence of effect, but no clear recommend from systematic review |
First-line treatment | ||
4 |
Severe nonuniform disease (e.g., aspiration syndromes) |
Level 4–5 strong uncontrolled and anecdotal evidence, small RCT showed strong trend |
5 |
Pulmonary hypoplasia (e.g., congenital diaphragmatic hernia) |
Level 4–5, but physiologically sound |
6 |
Severe chest wall restriction and/or upward pressure on the diaphragm due to abdominal distension |
Level 3 evidence (cohort studies, supported by animal research) |
7 |
Severe respiratory failure and PPHN (ECMO and iNO candidates) |
Level 4–5 strong uncontrolled and anecdotal evidence, small RCT showed strong trend |
8 |
Impaired hemodynamic status |
Level 3 evidence |
9 |
Nonuniform disease – BPD |
Level 4 evidence (small cohort studies) |
10 |
Intraoperative use |
Level 4–5 |
19.1.2.1 Respiratory Distress Syndrome and Other Surfactant Deficiency States
There are sound pathophysiologic reasons why patients with respiratory distress syndrome (RDS) and congenital pneumonia may benefit from high-frequency jet ventilation. Their lungs are prone to atelectasis and the time constants are short, thus lending themselves well to ventilation at high frequencies with little risk of air trapping. The lungs are relatively uniformly involved and can be effectively recruited (made more homogeneously inflated) with appropriate lung recruitment strategies. The rationale behind all HFV modes is that the use of small tidal volumes at high frequencies allows more uniform lung inflation and causes less damage to severely noncompliant lungs than do the larger tidal volumes of conventional ventilation. While more extensive animal data are available for HFOV, there are several animal studies that document less lung injury with HFJV, compared to conventional ventilation (Keszler et al. 1982; Quan et al. 1984; Sugiura et al. 1990), as well as the ability to use lower airway pressures (Boros et al. 1989).
19.1.2.1.1 Clinical Studies: Rescue Therapy
As with most new therapies, HFJV in the clinical setting was initially used in late rescue mode. Although many of the early studies included infants whose lung disease was complicated by air leak (discussed in detail later in the chapter), their underlying disease was severe uniform atelectatic disease, mostly RDS. Those early studies demonstrated apparent benefits with improved gas exchange and better survival in these moribund infants, relative to expectations (Boros et al. 1985; Spitzer et al. 1989). Subsequently, Baumgart et al. described a series of potential candidates for extracorporeal membrane oxygenation (ECMO) who were treated with HFJV also with apparently encouraging results (Baumgart et al. 1992). Infants with homogeneous lung disease (RDS or pneumonia) responded much better than those with meconium aspiration or lung hypoplasia. A small randomized trial by Carlo et al. failed to confirm improved survival (Carlo et al. 1990). The investigators used a device that is not commercially available and a low-pressure strategy. The study employed a sequential analysis design and was only powered to detect extreme improvement in survival from 15 to 85 %. Davis et al. suggested that the combination of HFJV and exogenous surfactant may be more effective than either technique alone, based on a series of 28 infants with poor initial response to exogenous surfactant (Davis et al. 1992).
Despite the lack of well-controlled clinical trials, many clinicians continue to use HFJV in rescue mode, based on available literature and personal experience (the response to HFJV is often quite dramatic and unequivocal). The time for controlled clinical trials of rescue HFJV has passed, lack of level 1 evidence notwithstanding, high-frequency ventilation has become a de facto standard of care in infants with severe RDS failing conventional ventilation, in part because lung volume recruitment is more easily achieved with high-frequency ventilation. The trend has shifted over time from late rescue to early rescue, as discussed above. Today, most clinicians will initiate HFJV long before the infant is in extremis, using high inflation pressure requirement or CO2 retention as the criteria for HFJV.
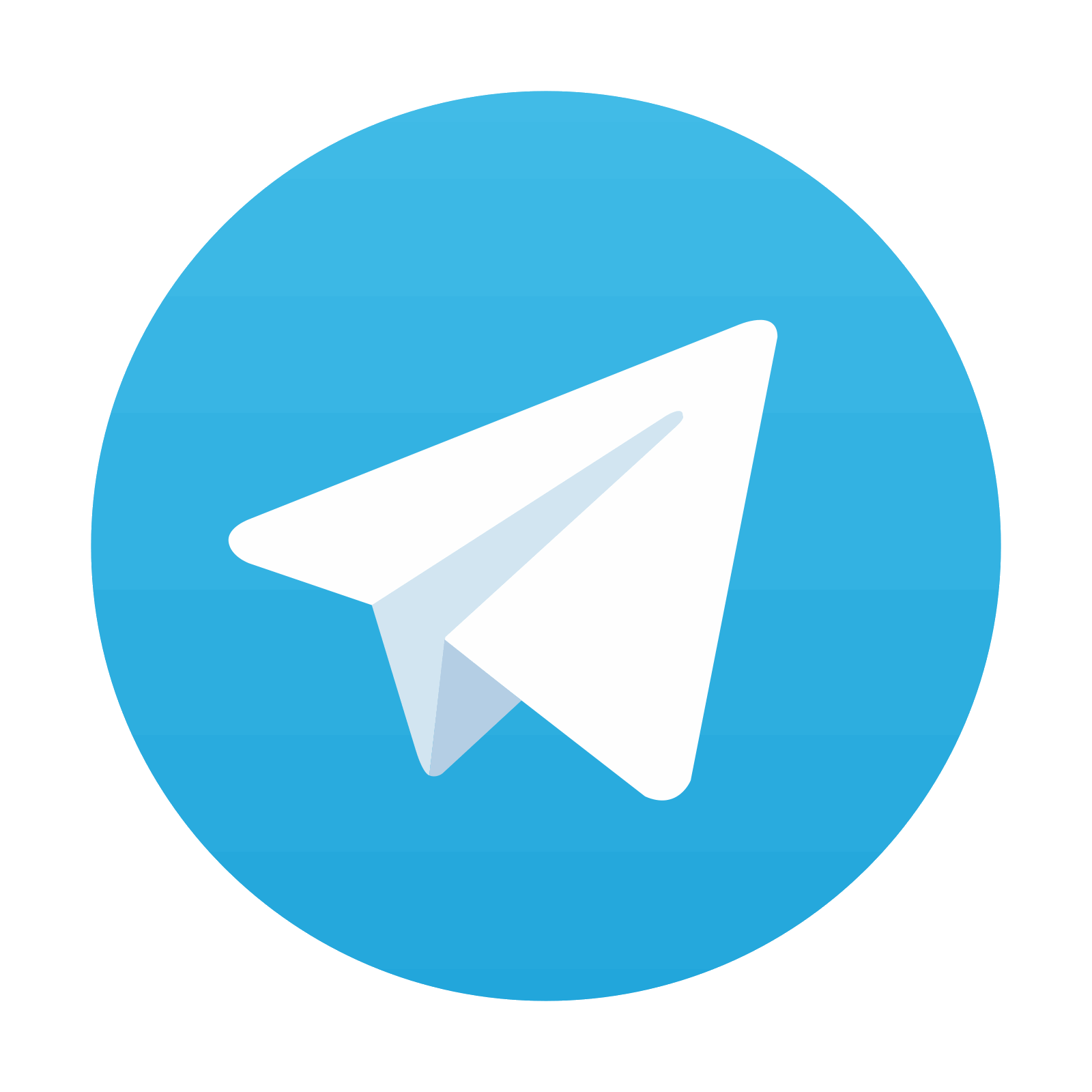
Stay updated, free articles. Join our Telegram channel
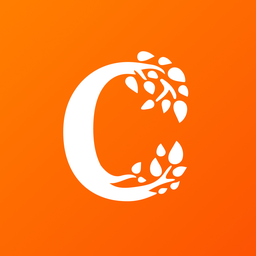
Full access? Get Clinical Tree
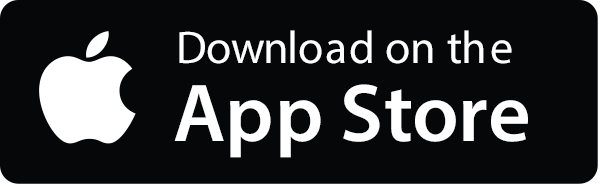
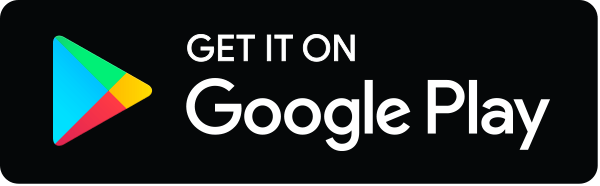