Ontogeny of the Immune System
INTRODUCTION
The fetus and neonate are more vulnerable than older children and adults to severe infection with pathogens, including pyogenic bacteria, fungi, viruses, and intracellular protozoa.1 Although this vulnerability indicates substantial limitations in innate and adaptive immunity in prenatal and early postnatal life, the mechanistic basis for these is only partially understood. Hematopoietic stem cell transplantation also provides compelling evidence for impairment of neonatal T-cell and natural killer (NK) cell immunity: Allogeneic hematopoietic cell transplantation with cord blood is associated with a significantly lower risk of acute graft-vs-host disease—a disease that is mainly mediated by donor-derived naïve T cells—compared to bone marrow and peripheral blood transplants containing adult T cells.2
DENDRITIC CELLS AND ANTIGEN PRESENTATION
CD11c+ dendritic cells (DCs) are myeloid-derived cells that participate in antigen presentation to T cells and B cells and also produce critical cytokines that shape adaptive immune responses. For example, the cytokines interleukin (IL)-12 p70, a heterodimer of the IL-12/IL-23 p40 subunit and IL-12 p35, and IL-15, which are produced by DCs, not only provide early innate immune protection but also promote the development of an adaptive immune response dominated by T helper 1 (Th1)–type effector CD4 T cells that produce interferon (IFN)-γ. Plasmacytoid dendritic cells (pDCs) are a distinct DC population that, at rest, lacks the characteristic dendrite-like protrusions of CD11c+ DCs and is found in lymphoid tissue, the circulation, and certain sites of tissue inflammation (eg, the skin in herpes simplex virus [HSV] infection). Activated pDCs are an important source of type I IFNs, which include multiple types of IFN-α and a single type of IFN-β. These type I IFNs are an important source of early and systemic innate antiviral immunity and enhance the later adaptive immune response, including Th1 differentiation from naïve CD4 T-cell precursors. DCs are activated by their recognition of conserved structures of microbial pathogens by toll-like receptors (TLRs), C-lectin-type receptors (CLRs), retinoic acid inducible gene-I (RIG-I)-like receptors (RLRs), and nucleotide binding domain and leucine-rich repeat-containing receptors (NLRs)3 as well as other stimuli.
DCs in Tissues
The colonization of CD11c+ DCs in extralymphoid and lymphoid tissues is developmentally regulated and occurs independently of exposure to inflammatory mediators. Immature CD11c+ DC lineage cells are found in the interstitial regions of solid organs, including the kidney, heart, pancreas, and lung, but not the brain, by 12 weeks of gestation; their numbers progressively increase through 21 weeks’ gestation.3 Epidermal DC-like cells that express the human MHC (major histocompatibility complex) class II isotype, HLA-DR (human leukocyte antigen DR), are found in the skin earlier, at 7 weeks’ gestation, and are probably derived from CD45+ HLA-DR+ cells that enter the epidermis, extensively proliferate, and then acquire the characteristic features of Langerhans DCs, including CD1c, langerin, and CD1a in a stepwise manner.4
Circulating and Monocyte-Derived DCs
Most phenotypic and functional studies of primary human DCs have for practical reasons analyzed circulating DCs, although a major caveat is whether these results also apply to tissue DCs.3 Circulating CD11c+ DCs are typically CD11chigh and HLA-DRhigh but are lineage-negative (Lin–), that is, lacking markers for other cell lineages, such as T cells, monocytes/macrophages, B cells, NK cells, granulocytes, and erythroid cells. Human pDCs are CD11clowLin– and express high levels of CD123 (the IL-3 receptor α -chain) and blood-derived dendritic cell antigen (BDCA)-2 (CD303 or C-type lectin-like receptor 4C [CLEC4C]), a type II transmembrane C-type lectin. Cord blood and adult peripheral blood have similar concentrations of CD11c+ DCs that are also phenotypically similar.3 An exception is CD86 (B7–2), a protein that engages CD28 on the T cell and provides it costimulation and that is lower on cord blood CD11c+ DCs. In contrast, the concentration of pDCs in cord blood is significantly higher than in adult peripheral blood and gradually declines after birth.3
Circulating neonatal DCs have selective limitations in the TLR-mediated upregulation of expression of molecules involved in T-cell costimulation by CD28 engagement (CD80 and CD86) and other DC–T-cell interactions (HLA-DR and CD40) compared to those of the adult.3 For example, cord blood CD11c+ DCs have reduced CD40 expression after exposure in vitro to ligands for TLR-2/6 (Mycoplasma fermentans), TLR-3 (polyinosine-cytosine (I:C), TLR-4 (lipopolysaccharide [LPS]), and TLR-7 (imiquimod) and have reduced CD80 in response to TLR-3 and TLR-4 ligands. In contrast, CD11c+ DCs of neonates and adults similarly increase CD40 in response to GU-rich single-stranded RNA, a TLR-8 ligand, and MHC class II and CD86 after stimulation with TLR-3 and TLR-4 ligands. The pDCs of cord blood and adult peripheral blood also similarly upregulate HLA-DR, CD80, and CD86 after stimulation with TLR-9 ligands, such as DNA phosphothioate oligonucleotides lacking methylation of CpG residues (CpG DNA).3
Th1 immunity may be particularly limited in the neonate and young infant compared to older individuals because of decreased IL-12 p70 production by CD11c+ DCs, such as in response to LPS plus IFN-γ.1 However, this limitation is likely pathogen dependent, as decreased IL-12 production by cord blood CD11c+ DCs is similar to adult CD11c+ DCs after stimulation with certain gram-positive and gram-negative bacteria or meningococcal outer-membrane proteins.1 Circulating DCs from cord blood can also stimulate allogeneically (ie, in a MHC mismatched context) cord blood T cells in vitro,1 but it is unclear if they are as effective as adult DCs in promoting Th1 differentiation under these conditions.
The production of type I IFNs by cord blood CD11c+ DCs and pDCs is also reduced compared to those of adult peripheral blood and could contribute to the limited ability of the neonate to control pathogens such as HSV. For example, IFN-α production by cord blood CD11c+ DCs in response to a TLR-3 ligand and by cord blood pDCs in response to TLR-9 ligands—such as live or inactivated HSV or CpG DNA—is reduced compared to adult DCs.3 This decreased production of type I IFNs is not attributable to diminished TLR-9 expression by cord blood pDCs but appears to be due to reduced nuclear translocation of interferon regulatory factor 7 (IRF7), a transcription factor that is essential for type I IFN gene expression.3
A number of studies have compared the function and phenotype of monocyte-derived dendritic cells (MDDCs), which are produced by culturing monocytes from cord blood or adult peripheral blood in vitro with granulocyte-macrophage colony-stimulating factor (GM-CSF) and IL-4; these MDDCs are typically evaluated for function after their activation by incubation with tumor necrosis factor (TNF)-α or other stimuli. Although cord blood MDDCs have reduced IL-12 p70 expression after stimulation, it is unclear if these and other functional and phenotypic differences apply to fetal and neonatal DCs of the tissues. Gene expression profiling analysis suggested that MDDCs may best model inflammatory DCs, which are generated from monocytes in vivo at sites of marked tissue inflammation, such as during Th1-driven reversal reactions of infection with Mycobacterium leprae.3 It remains unclear regarding the extent these inflammatory DCs are generated in the fetus and neonate in response to infection or other proinflammatory stimuli.
Postnatal DC Maturation
HLA-DR expression and CD80 expression induced by LPS (a TLR-4 ligand), which, as noted, is significantly reduced in cord blood CD11c+ DCs, reaches the levels of circulating adult CD11c+ DCs by 3 months of age.5 In a longitudinal study of infants from the Gambia, TNF-α, IL-6, and IL-12/IL-23 p40 expression by CD11+ DCs in response to LPS were significantly greater at 1 year and 2 years of age than at birth or compared to their expression by these cells in adult peripheral blood.6 In contrast, CD11c+ DCs of newborns, 1-year-olds, and 2-year-olds all had higher levels of production of these cytokines in response to a TLR-2 agonist (PAM 3Cys) and a TLR-7/8 agonist (3M-003). For pDCs, adult levels of HLA-DR and CD80 expression induced by a TLR-9 agonist (CpG DNA) were not achieved until 6–9 months of age.6 The production by pDCs of TNF-α and IL-6 and in response to either TLR-7/8 or TLR-9 agonists at 1 year of age was similar to those of adult pDCs. Thus, the maturation of DC function after birth is not uniform for all functions but rather occurs in a TLR-specific manner. The maturation of responses to non-TLR ligands has largely not been explored.
T CELLS
Ontogeny of Thymic Development
Initial colonization of the fetal thymus anlage by prothymocytes occurs at 8.5 weeks of gestation. This is followed by dramatic increases in thymic cellularity during the second and third trimesters. Transient thymic involution, with a prominent loss of cortical double-positive (CD4highCD8high) thymocytes, appears to occur at the end of the third trimester due to a prenatal surge in the circulating glucocorticoid levels.1 This involution is followed by recovery of thymocyte cellularity at 1 month of age. Peak thymocyte cellularity in relation to body size and, presumably, output of recent thymic emigrants (RTEs), occurs between 6 and 12 months of age.1,7
Repertoire of αβ-T-Cell Receptors
T-cell receptor diversity is generated by the process of V(D)J recombination in developing thymocytes and involves the joining variable (V), diversity (D), and joining (J) segments in the case of the T-cell receptor (TCR)-β chain and V and J segments in the case of the TCR-α chain. The usage of TCR-β D and J gene segments in the thymus is initially less diverse than subsequently. The complementarity determinant region 3 (CDR3) region of the TCR-β chain transcripts, which is encoded at the 3′ end of the V segment and the adjacent D and J segments, is also reduced in length and sequence diversity between 8 and 15 weeks of gestation. This is likely due to decreased activity of the terminal deoxytransferase (TdT) enzyme, which performs random N-nucleotide addition during V(D)J recombination. An impact of limitations in αβ-TCR diversity on the ability of the fetus to respond to congenital infection would be expected to be most pronounced in the first trimester of pregnancy, as intrathymic TdT activity, CDR3 length, and V segment diversity by the second trimester are similar to that of postnatal thymic tissue.1 A low-resolution analysis (ie, not direct DNA or messenger RNA [mRNA] sequencing) of the TCR repertoire expressed on cord blood T cells indicates that the diversity of TCR usage and CDR3 length are similar to that of antigenically naïve T cells in adults and infants. Together, these observations suggest that the functional preimmune repertoire is well formed by birth.
Signal joint TCR excision circles (sjTRECs) are generated as a part of TCR-α gene rearrangement of thymocytes and may persist for long periods in T-lineage cells that do not proliferate. sjTREC levels in cord blood are relatively high compared to adult peripheral blood, consistent with the expected predominance of naïve (CD45RAhighCD45R0low) T cells and RTEs in cord blood (see “Fetal T-Cell Development and Phenotype” section below).1,8
Fetal T-Cell Development and Phenotype
By 14 weeks’ gestation, CD4 and CD8 T cells are found in the fetal circulation, liver, and spleen, and T cells are detectable in lymph nodes. The percentage of circulating T cells increases during the second and third trimesters of pregnancy until about 6 months of age, followed by a gradual decline to adult levels. The ratio of CD4 to CD8 T cells in the circulation is high during fetal life (about 3.5) and gradually declines with postnatal age.
Most circulating CD4 T cells in the term and preterm neonate and in the second- and third-trimester fetus express a CD45RAhighCD45R0lowCCR7+CD25–CD95– surface phenotype, which is similar to that of antigenically naïve CD4 T cells of the adult circulation. However, second-trimester naïve CD4 T cells have high basal levels of Foxp3, a key transcription factor for regulatory T-cell (Treg) development and function, and, upon allogeneic stimulation, are highly skewed toward differentiation into Tregs, which potently suppress effector T-cell function. In contrast, adult peripheral blood naïve CD4 T cells have lower basal levels of Foxp3 and preferentially differentiate into effector cells with allogeneic stimulation.9 Tregs are also more abundant in fetal peripheral lymphoid tissue compared to that of the adult.10 This tendency for Treg skewing by fetal CD4 T cells appears to be a characteristic of T cells that are derived from fetal hematopoietic stem cells (HSCs) rather than adult HSCs and is likely an important mechanism for fetal-maternal tolerance.9 It remains unclear at what age this fetal HSC program of T-cell differentiation is replaced with an adult-type HSC program favoring effector cell rather than Treg differentiation.
Protein tyrosine kinase 7 (PTK7), a surface protein and member of the receptor tyrosine kinase superfamily, identifies RTEs among human adult naïve CD4 T cells and is also expressed uniformly at high levels by cord blood T cells and postnatal thymocytes.8 These observations are consistent with the fetal and neonatal peripheral T-cell compartment being enriched in RTEs, an idea that is also supported by the high level of thymic cellularity in the newborn and young infant.7 Cord blood T cells may be more prone than adult naïve T cells to undergo homeostatic proliferation because of a greater sensitivity to the mitogenic effects of IL-7, and this may also be a reflection of their enrichment in RTEs, which also have this propensity.1,8 In contrast, CD38, a cell surface ectoenzyme involved in intracellular calcium mobilization, is found on virtually all peripheral fetal and neonatal T cells as well as fetal and postnatal thymocytes but is absent on adult naïve T cells, including adult RTEs.1,8 Together, these observations suggest that peripheral T cells in the fetus and neonate have a distinct thymocyte-like immature phenotype beyond that attributable to their enrichment in RTEs.
CD4 T-Cell Responses
Herpesvirus Infections
A striking limitation in neonatal T-cell immunity is the reduced and delayed HSV-specific CD4 T-cell cytokine (IL-2, IFN-γ, and TNF-α) production and proliferation in neonates compared to adults after primary HSV infection.11 CD4 T-cell help for HSV-specific antibody production is also likely reduced in neonates.1 Older infants and young children also have reduced CD4 T-cell immunity (antigen-specific IL-2, IFN-γ, and CD40-ligand [CD154] expression) after primary infection with cytomegalovirus (CMV), another herpesvirus, compared to adults.12 This reduced CD4 T-cell response is associated with persistent CMV shedding in the urine and saliva of young children following primary infection.12 This reduced response also likely applies to the neonate and young infant with congenital, perinatal, or postnatally acquired CMV infection, all of whom persistently shed CMV for years following its acquisition.
Vaccines
The robustness and quality of the CD4 T-cell response of the neonate and young infant to live vaccines varies with the particular agent. BCG vaccination at birth is as effective as vaccination at 2 or 4 months of age for inducing mycobacterial-specific CD4 T-cell proliferation and IFN-γ production and is not associated with increased Th2 skewing (ie., IL-4, IL-5, or IL-13 production) and reduced levels of IFN-γ.1 However, the Th1 responses of infant BCG vaccine recipients have not been directly compared with those of older children and adults. In contrast to BCG, newborns given oral poliovirus vaccine (OPV) at birth, 1, 2, and 3 months of age have lower OPV-specific CD4 T-cell proliferation and IFN-γ production compared to adults who were immunized later in childhood.1 Interestingly, the neonate’s and infant’s antibody titers are higher than those of adults, suggesting that CD4 follicular T-cell help for B cells is not impaired by early OPV immunization. OPV may be less effective at inducing a Th1 response than BCG in neonates and young infants because of its limited replication (BCG vaccination results in persistent infection), its site of inoculation, or a more limited ability to stimulate antigen-presenting cells (APCs) in a manner conducive to Th1 immunity. In contrast to BCG, the administration of pertussis vaccine to neonates may result in a skewing of pertussis antigen-specific T cells toward a Th2 cytokine profile.13
Mechanisms for Reduced Immunity
Normal CD4 T-cell activation by engagement of the αβ -TCR and the CD28 molecule on the T-cell surface results in the activation of transcription factors, including NFAT (nuclear factor of activated T cells), AP-1(activated protein-1), and NF-κ B (nuclear factor-κ B), resulting in the de novo transcription of genes such as IL-2 and CD154. IL-2 promotes CD4 T-cell proliferation in an autocrine and paracrine fashion. CD154 engages CD40 on CD11c+ DCs to increase their surface expression of CD80 and CD86 and their production of IL-12 p70. In addition to the limitations in DC function discussed, several other potential mechanisms intrinsic to the neonatal CD4 T cell may limit its ability to expand into memory/effector Th1 cells. These impairments include reduction in neonatal CD4 T-cell NFAT proteins, which is mediated by microRNA-184,14 IL-2 and CD154 expression,15 and IL-12p70-induced activation of signal transducer and activator of transcription 4 (STAT4) by tyrosine phosphorylation,15
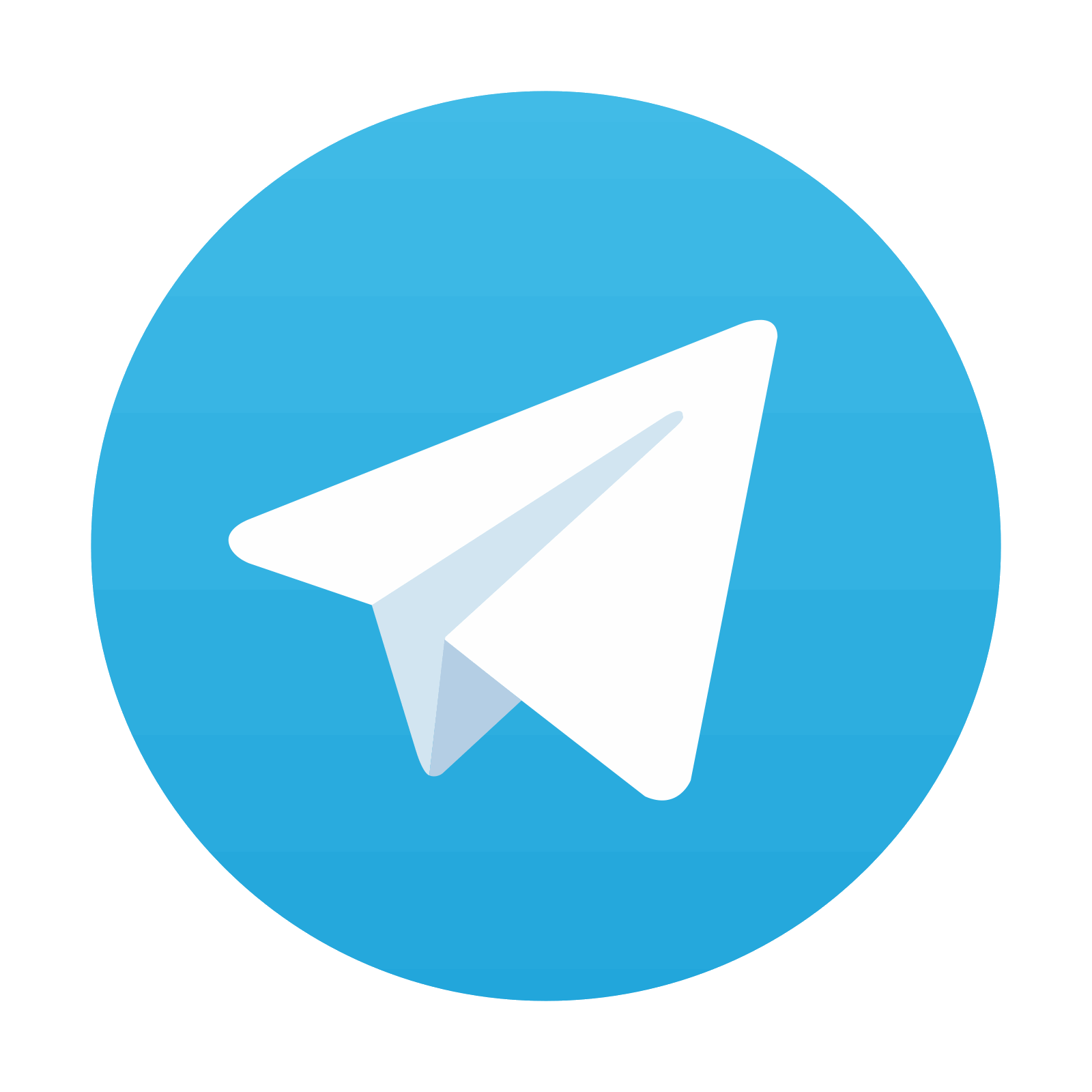
Stay updated, free articles. Join our Telegram channel
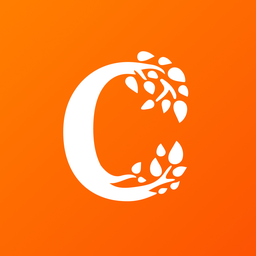
Full access? Get Clinical Tree
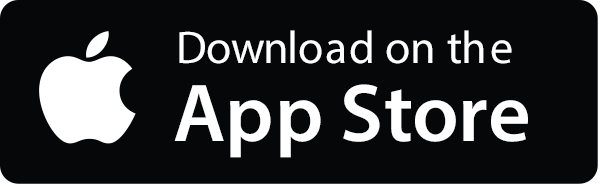
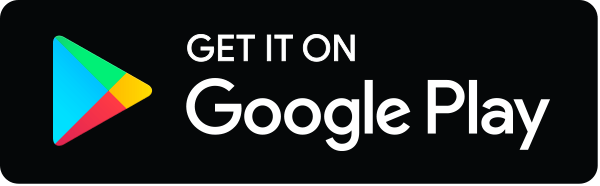