Fluids and Electrolytes
Evan P. Nadler
Edward M. Barksdale Jr.
New York University School of Medicine, New York, New York 10016.
Department of Surgery, University of Pittsburgh, Department of Pediatric Surgery, Children’s Hospital of Pittsburgh, Pittsburgh, Pennsylvania 15213.
The management of fluid and electrolytes in surgical patients is a dynamic process that requires not only a thorough understanding of the pathogenesis of the disease, but also a clear concept of the patient’s physiologic status. Nowhere is this more critical than in the care of infants and children. Neonates especially have unique characteristics that must be taken into account to provide optimal fluid management after surgery. This chapter discusses fluid and electrolyte management and postoperative intervention in pediatric patients, with special emphasis on the newborn. Disorders in electrolyte balance and strategies for treating these abnormalities are also addressed. The goal of this chapter is to outline the important physiologic considerations in the pediatric surgical patient and to give the pediatric surgeon direction in caring for these children.
Any discussion of fluid and electrolyte management must begin with the basic concept that net balance is equivalent to the amount of fluid and electrolytes lost subtracted from the total intake during a specific reference period. In most surgical patients, intake is controlled by the caregiver and should be governed by calculating expected losses and the homeostatic requirements of each individual child. Thus, fluid and electrolyte management in the surgical patient can be broken down into three major components: total body fluid and its composition, renal excretion and insensible losses, and other postoperative deficits, including stoma output and third-space losses.
TOTAL BODY FLUID COMPOSITION
Body Water and Fluid Distribution
Water is the major contributor to total body weight and accounts for nearly 60% of body weight in adults. However, total body water (TBW) comprises a much higher proportion of the total body weight in neonates. Estimates for fetal TBW are greater than 90% of total body weight early in gestation, close to 80% at 32 weeks’ gestation, and approximately 75% at term (1). There is an additional decrease in TBW over the first few days of life in the term infant, and adult levels are generally reached by 1 year of age. However, the premature infant has a TBW similar to that of the fetus and must accomplish fluid redistribution and diuresis in a short period (days to weeks) after birth rather than more gradually (weeks to months) in utero. Neonates with intrauterine growth retardation (IUGR) have similar body water distribution as premature infants of similar birthweight (2) (Fig. 6-1). Infants who fail to unload fluid effectively or who receive excess fluid during normal postnatal diuresis may be at increased risk for developing a patent ductus arteriosus, chronic lung disease, and even necrotizing enterocolitis (3,4).
The TBW is usually divided into two compartments, intracellular and extracellular fluid. The intracellular fluid (ICF) constitutes approximately two-thirds of the TBW in the adult, whereas the extracellular fluid (ECF) is responsible for the other one-third. The ECF is further subdivided into interstitial fluid (75% of ECF) and plasma volume (25%). Fluid distribution is markedly different in the fetus and infant. A series of studies by Friis-Hansen suggested that ICF comprises approximately 25% of the total body weight in a 20-week gestational fetus, which gradually increases to 33% at term, unless the process is interrupted by premature birth or IUGR (5,6,7,8). Adult levels (40%) are generally reached by 3 months of age. In the fetus, ECF generally decreases with the TBW as gestation progresses. It accounts for nearly 60% of the total body weight in the 20-week fetus, 45% at term, and approximates the adult level (20%) by 1 year of age. Thus, the newborn has a disproportionate ratio of fluid in the extracellular space compared with the intracellular space until at least the end of the first year of life and, therefore, may be more susceptible to fluid overload.
Electrolyte Distribution
The appropriate distribution of water in the intracellular and extracellular spaces is regulated by osmotic gradients. Because the cell wall is a semipermeable membrane, it allows for the free passage of water in response to changes in ion concentration on either side of the membrane. The major extracellular cation is sodium; potassium is the major intracellular cation. The major extracellular anions are chloride and bicarbonate; phosphates and nondiffusable proteins predominate intracellularly. Thus, significant changes to any one of the principle ions, most commonly the extracellular ions, may cause alterations in fluid distribution and subsequently cell and organ function.
Sodium balance is the key regulator of ECF volume. However, it is the total body sodium, not merely the serum sodium, that is responsible. The majority of total body sodium is in the bone, which usually contains nearly 25 meq per kg. In addition, there is approximately 17 meq per kg of sodium in the interstitial fluid, 6.5 meq per kg in plasma, and about 1.5 meq per kg intracellularly. The rest of the total body sodium is located within connective tissue and cartilage, totaling about 10 meq per kg. Thus, the sodium content for the entire body approaches 60 meq per kg. The fetus has a higher proportion of ECF than the adult and, therefore, the total body sodium is closer to 90 meq per kg. The body strives to keep intracellular sodium constant, using active transport of sodium out of the cells via a sodium-potassium ATPase. This pump keeps intracellular sodium close to 10 meq per L at the expense of extracellular sodium. Changes in extracellular sodium may or may not be reflected in serum levels, which are usually 135 to 145 meq per L regardless of patient age.
Sodium intake is usually via enteral nutrition, although in surgical patients it is often delivered via intravenous fluids or hyperalimentation. Daily needs are approximately 3 meq per kg per day. Absorption occurs in the jejunum via a sodium-potassium ATPase mechanism. Daily losses of sodium are mostly through urine, sweat, and feces. The kidney regulates sodium loss in response to a variety of host factors with the goal of maintaining appropriate fluid balance. This is discussed further in the following section on renal function. Sweat content may vary from 5 to 40 meq per L of sodium. Levels may be significantly higher in patients with cystic fibrosis due to dysfunctional sodium and chloride transport (9,10). Fecal losses are usually minor; however, they can be significant in patients with diarrhea or high output ostomies (11,12,13).
Potassium serves a critical function in maintaining the resting cell membrane potential. It is generally unbound inside the cell and contributes to the osmotic gradient in electrically active cells. A membrane-bound sodium-potassium ATPase exchanges three intracellular sodium ions for two extracellular potassium ions, thus maintaining a net negative balance of positive ions. The ATPase in conjunction with the nondiffusable proteins inside the cell results in the-90 mV resting potential of most cells. Passive transport of potassium may occur through voltage-gated potassium channels, which are impermeable to sodium. Therefore, variations in serum potassium levels more than sodium levels, result in altered resting potentials and associated cell function. An increase in extracellular potassium results in an influx of positive ions into the cell, a relative depolarization and excitability of the cell. Hypokalemia results in an efflux of potassium out of cell and hyperpolarization, which renders the cell less likely to generate an action potential.
Similar to sodium, potassium is either ingested as part of the daily diet or given to surgical patients via intravenous fluids. Approximately 2 meq per kg per day are required to maintain homeostasis. Most of the potassium is absorbed in the upper portions of the gastrointestinal
tract, with a minor amount of potassium absorbed in the distal colon. Extracellular potassium is governed not only by total body potassium, but also by hormonal influences on the kidney as well as other organ systems. Insulin, catecholamines, thyroid hormone, and aldosterone all influence sodium-potassium ATPase activity (14). Importantly, insulin and catecholamines result in a net influx of potassium into cells and a decrease in overall serum levels. Similarly, bicarbonate administration can be used to drive potassium into the cells in exchange for hydrogen ions. Metabolic acidosis has the opposite effect. The kidney is responsible for nearly all potassium excretion. This is discussed in detail in the following renal function section. There is minimal loss of potassium in the sweat or feces, although similar to sodium, fecal losses may be significant in patients suffering from diarrhea or high-output stomas (11,13).
tract, with a minor amount of potassium absorbed in the distal colon. Extracellular potassium is governed not only by total body potassium, but also by hormonal influences on the kidney as well as other organ systems. Insulin, catecholamines, thyroid hormone, and aldosterone all influence sodium-potassium ATPase activity (14). Importantly, insulin and catecholamines result in a net influx of potassium into cells and a decrease in overall serum levels. Similarly, bicarbonate administration can be used to drive potassium into the cells in exchange for hydrogen ions. Metabolic acidosis has the opposite effect. The kidney is responsible for nearly all potassium excretion. This is discussed in detail in the following renal function section. There is minimal loss of potassium in the sweat or feces, although similar to sodium, fecal losses may be significant in patients suffering from diarrhea or high-output stomas (11,13).
Chloride balance is maintained in parallel with sodium balance. Chloride is the major anion in the ECF, and it moves between the cell and extracellular space mostly via passive diffusion. Thus, it follows the electrochemical gradient created by the passage of sodium into and out of cells. Its excretion by the kidney is critical to the maintenance of proper acid–base status. The administration of massive amounts of chloride, as in hypovolemic resuscitation using normal saline, may result in hyperchloremic metabolic acidosis. Similarly, increased resorption of chloride in the kidney results in renal tubular acidosis. Significant chloride losses from protracted vomiting in patients with pyloric stenosis may result in metabolic alkalosis and must be corrected prior to surgery.
RENAL EXCRETION AND INSENSIBLE LOSSES
Postnatal Kidney Function
Urine produced by the kidney is responsible for the majority of fluid and electrolyte losses. In the term infant, urine output is low on the first day of life, and gradually increases as intake increases and kidney function improves. Appropriate renal function depends on the glomerular filtration rate (GFR) and the tubular reabsoptive capacity. There are significant changes in both of these processes as the fetus develops.
Lorenz and colleagues illustrated that three phases of fluid and electrolyte homeostasis occur. This appears to be true in low-birth-weight (preterm) infants and may also be applicable to term newborns (15,16,17).
The first phase is termed the prediuretic phase and is associated with low urine output, often less than 1 cc per kg per day. The prediuretic phase usually starts immediately after birth and lasts for the first 24 hours of life. Excess fluid administration at this time may result in fluid overload if the low urine output is mistakenly interpreted as hypovolemia. The GFR at this time is low, especially in the premature infant, but then gradually increases postnatally despite other medical conditions that may be relevant (18). GFR is fundamentally determined by the renal perfusion pressure and the renovascular resistance. The newborn infant has a relatively low percentage of cardiac output directed to the kidney (∼6%) compared with the adult kidney (∼25%). This is the result of relatively high vascular resistance, which begins to decrease after the first postnatal day, and accounts for the relatively low GFR seen in the prediuretic phase. In the first week of life, GFR increases up to fivefold, and provides for the increase in urine output and renal function seen in the second phase of postnatal fluid balance, the diuretic phase.
Urine output of up to 7 cc per kg per day marks the diuretic phase. It usually begins around the second day of life, and lasts for 48 to 72 hours. Due to the relatively high urine output, large volume and sodium losses may be encountered. The diuresis and natiuresis may be accomplished even in the face of fluid and sodium administration (16). As previously stated, neonates who do not achieve negative fluid balance during the diuretic phase of homeostasis are at increased risk for patent ductus arteriosis, congestive heart disease, chronic lung disease, and necrotizing enterocolitis. By day of life 4 or 5, urine output and sodium excretion begin to vary based on the fluid and electrolytes administered or ingested. This time period is referred to as the postdiuretic phase. After the first week of life, the unloading of excess prenatal water and sodium is usually accomplished. The GFR then continues to increase rapidly in proportion to body weight until about 3 months of age. This is followed by a slower increase in GFR until adult levels are reached by about 2 years of age.
Inability to concentrate the urine in the proximal and distal convoluted tubules of the kidney is the second factor that contributes to the unique renal function in the early postnatal period. The neonatal kidney has only about one-half the concentrating capacity as the adult kidney. The serum osmolality is generally governed by antidiuretic hormone (ADH) synthesis by the hypothalamus and secretion by the posterior pituitary. When the serum osmolality exceeds 280 mOsm, the pituitary secretes ADH. In contrast, when serum osmolality is below 275 mOsm, ADH secretion is virtually absent. ADH influences the collecting tubules to absorb water into the hypertonic interstitial fluid without significant electrolyte transport. Thus, the resulting urine is concentrated. However, despite the fact that ADH synthesis seems to be intact in the term and preterm neonate, the sensitivity of the newborn renal tubules to ADH is blunted compared with the adult (19,20). The contrary is true with regard to dilution of the urine. Free water clearance by the newborn is well above the capacity of the adult kidney (21). Excess fluid administration can still overwhelm the kidney’s ability to produce
dilute urine and achieve the appropriate postnatal diuresis, despite the increased capacity to do so.
dilute urine and achieve the appropriate postnatal diuresis, despite the increased capacity to do so.
The renal tubules in the newborn are also immature when it comes to sodium excretion. The filtered sodium load is dependent on the GFR, which in turn is regulated by overall fluid status. Volume contraction leads to a decreased GFR and results in decreased sodium filtration (22). In response to volume contraction, the juxtaglomerular apparatus of the kidney responds by releasing renin and stimulating the renin-angiotensin-aldosterone axis. Aldosterone stimulates exchange of sodium for potassium in the cortical collected ducts and an increase in sodium loss. Responsiveness to aldosterone is somewhat reduced in the term infant when compared with the adult, but may even be further suppressed in the premature infant (23). The blunted response may be due to relatively low sodium-potassium ATPase activity (24).
The other major hormone involved in regulating sodium homeostasis is atrial natiuretic peptide (ANP). ANP is part of a sensitive cardiopulmonary regulatory system that leads to increased sodium excretion and volume contraction during periods of fluid overload (25). The sensitivity of this reflex decreases with maturation (26). In response to atrial stretch, ANP is released into the circulation. This increases GFR by dilating the afferent glomerular arterioles and thus increasing the filtered sodium load. In addition, ANP inhibits sodium resorption in the collecting ducts, providing a second mechanism for natiuresis. However, the contribution of ANP in the premature infant is unclear (27).
The fractional excretion of sodium (FeNa) is a useful tool in measuring sodium homeostasis and renal tubular function. It is calculated by multiplying the ratio of sodium in the urine and plasma by the ratio of creatinine in the plasma and urine.
FeNa = UNa/PNa × PCr/UCr × 100%
The normal adult resorbs greater than 99% of the sodium filtered. This results in an FeNa less than 1%. The same holds true for the term neonate. However, the normal premature kidney has a higher FeNa ranging from 3% to 9%, and this decreases as gestational age increases (28). An imbalance in the ratio of number of glomeruli to the number of tubules may be partially responsible for the decrease in sodium excretion (29). The inability to achieve maximal sodium excretion may also be in part due to poor expression and thus reduced activity of the sodium-potassium ATPase (30). As the kidney matures, the ratio of glomeruli to tubules decreases and the FeNa decreases in parallel. Damaged tubules are unable to excrete sodium and result in an elevated FeNa.
Most potassium excretion is also regulated by the kidney. The proximal convoluted tubule reabsorbs about two-thirds of the potassium filtered by the glomerulus. The ascending limb of the loop of Henle is responsible for the majority of the other one-third. As with sodium, a high GFR presents a high potassium load to the tubules and results in potassium excretion. Potassium secretion is dependent on electrical and chemical gradients, and is carried out in the distal convoluted tubules and collecting ducts. As long as urine output is high, the gradient between the urine and the cells in the tubules is maintained and secretion of potassium may ensue. Aldosterone induces potassium loss in the collecting ducts in exchange for sodium in periods where volume contraction exists and urine flow may be diminished.
It is imperative that all the aforementioned features of the neonatal kidney be kept in mind when resuscitating the neonate after operation. There is a physiologic need for the newborn to diurese, whereas surgical intervention requires volume expansion. The immaturity of the neonatal kidney complicates rehydration strategies and may result in electrolyte abnormalities if improper fluids are chosen for intravenous hydration or if the volume administered is inappropriate. These factors all contribute to the difficulties in managing the newborn surgical patient.
Insensible Water Losses
The second major mechanism for fluid loss in the newborn is through evaporative losses from the respiratory epithelium and through the skin, known as insensible losses. Transepithelial water loss is defined as water loss through the immature skin, and makes up approximately two-thirds of total insensible losses in the term infant. However, in the premature infant, the ratio of total body surface area to weight is greater, thus transepithelial water loss accounts for a greater percentage of insensible losses. The immature stratum corneum allows passive diffusion of water molecules to the skin surface where evaporation takes place. These water losses may be quite significant in the premature or small for gestational age infant, and it can take more than 4 weeks before a fully functional barrier is attained in the preterm infant (31). Studies by Hammarlund et al. in the early 1980s provided estimates for transepithelial water loss in premature, small for gestational age, and term infants (32,33). They found that mean transepithelial water loss was 110 g per kg per day for 25- to 27-week infants on the first day of life, whereas values for 28- to 30-week infants were less than one-half that of the smallest babies (Table 6-1). Term and near-term infants had negligible amounts of water loss. A simpler method for estimating transepithelial water loss has been used that suggests values of approximately 65 cc per kg per day in the first week of life for infants who weight less than 1,000 g, 55 cc per kg per day for infants between 1,000 and 1,250 g, and 40 cc per kg per day for infants between 1,250 and 1,500 g (34) (Table 6-2).
Phototherapy and nonionizing radiation from the radiant warmer have been reported to increase transepithelial
water loss, and their effect on overall loss is believed to be additive (35). Individually, phototherapy and nonionizing radiation can increase transepithelial water loss by as much as 50%. However, at least one more recent study questions whether phototherapy increases water loss via the stratum corneum (36). Increases in body temperature either by fever or by elevation in ambient temperature also add to insensible losses by as much as 7 mL per kg per day for each degree of elevation over 37°C (37,38).
water loss, and their effect on overall loss is believed to be additive (35). Individually, phototherapy and nonionizing radiation can increase transepithelial water loss by as much as 50%. However, at least one more recent study questions whether phototherapy increases water loss via the stratum corneum (36). Increases in body temperature either by fever or by elevation in ambient temperature also add to insensible losses by as much as 7 mL per kg per day for each degree of elevation over 37°C (37,38).
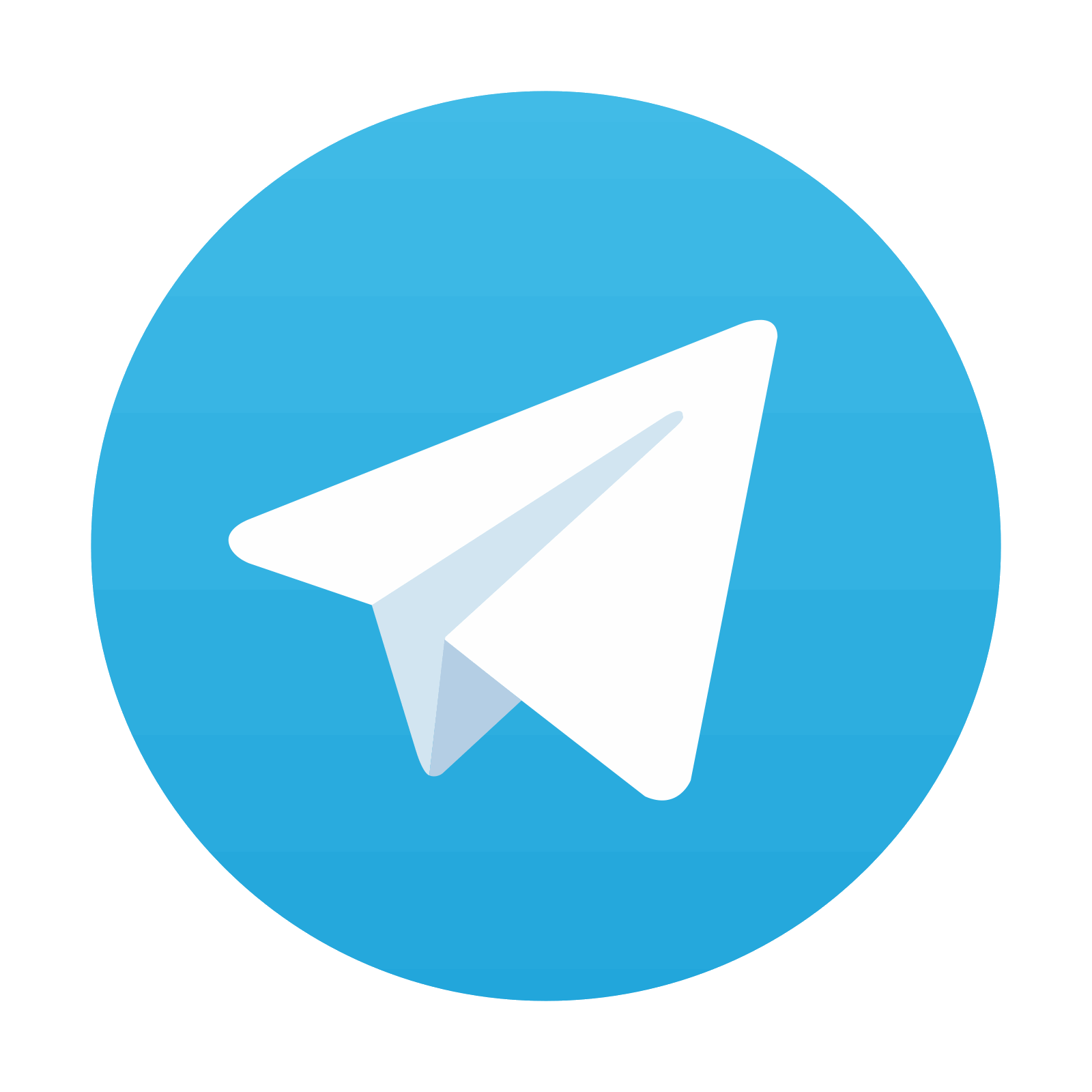
Stay updated, free articles. Join our Telegram channel
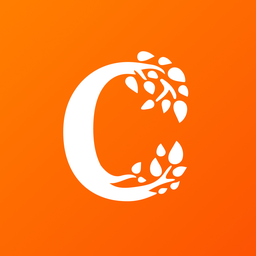
Full access? Get Clinical Tree
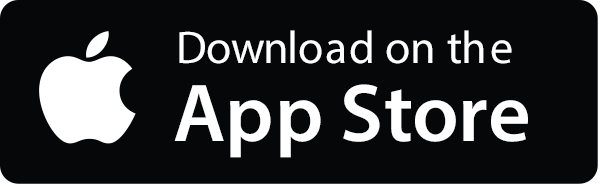
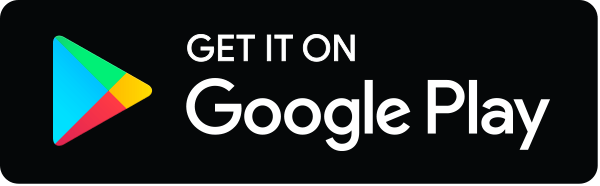