Total body water (TBW) encompasses extracellular (interstitial and plasma) and intracellular water. Early in fetal development, TBW is almost 95% of the total body weight. As the fetus grows, there is a decrease in the proportion of body weight represented by water (Figure 44-1).19 By birth, TBW represents approximately 75% of body weight in a full-term infant. The progressive decrease in TBW is caused primarily by decreases in the extracellular water compartment. Premature, particularly very premature, infants have a higher TBW content than term infants, and that increase is primarily extracellular water.23 During the first week of life, all healthy neonates experience a reduction in body weight. The major cause of this physiologic weight loss is a reduction in extracellular water.58 In the first 24 to 48 hours after birth, infants have decreased urine output, followed by a diuresis phase, with urinary losses of water and sodium in the first week of life, resulting in weight loss.37 Physiologic weight loss in the first few days of life in term and premature infants represents isotonic contraction of body fluids and seems to be part of a normal transitional physiologic process, although the precise mechanisms underlying this process are unclear. In term infants, this weight loss can be up to 10%. In very premature infants, weight loss can be up to 15%.60 Perturbations in this normal transitional physiology can lead to imbalances in sodium and water homeostasis. In ill term infants and premature infants, various factors (discussed subsequently) can lead to increased or decreased urinary or insensible water losses. Similarly, increased or decreased administration of intravenous fluids, with variable amounts of water and sodium, can have a significant impact on overall fluid balance. Healthy term neonates have basal sodium handling similar to that of adults, with a fractional excretion of sodium (FENA) of less than 1%, although a transient increase in FENA occurs during the diuretic phase on the second and third days of life.37 In premature infants, however, renal sodium losses are inversely proportional to gestational age, with FENA equaling 5% to 6% in infants born at 28 weeks’ gestation (Figure 44-2).62 As a result, preterm infants may display negative sodium balance and hyponatremia during the initial 2 to 3 weeks of life because of high renal sodium losses and inefficient intestinal sodium absorption.67 The mechanisms responsible for increased urinary sodium losses in preterm infants are multifactorial. The immature kidney exhibits glomerulotubular imbalance, a physiologic state that is present when the glomerular filtration rate (GFR) exceeds the reabsorptive capacity of the renal tubules. This imbalance is attributable to numerous factors, including a preponderance of glomeruli compared with tubular structures, renal tubular immaturity, large extracellular volume, and reduced oxygen availability.51 Decreased responsiveness to aldosterone is also characteristic of fetal and postnatal kidneys compared with adult kidneys, and results in a decrease in sodium reabsorption. Urinary sodium losses in preterm and term neonates may be increased in certain conditions, including hypoxia, respiratory distress, hyperbilirubinemia, acute tubular necrosis, and polycythemia. Pharmacologic agents such as dopamine, beta blockers, angiotensin-converting enzyme inhibitors, and diuretics may also increase urinary sodium losses in neonates. The abnormalities in sodium and water balance seen in premature infants are attenuated, to some degree, by prenatal steroid administration. Prenatal steroid treatment is associated with decreased insensible water loss, a decreased incidence of hypernatremia, and an earlier diuresis and natriuresis.44 This beneficial effect on water and sodium balance in infants with extremely low birth weight is thought to be mediated by maturation of the renal epithelial transport systems controlling fluid and electrolyte homeostasis. This system may not be as straightforward as previously believed, however, because vasopressin V2 receptors have been shown to be expressed in nephron segments other than the collecting duct, notably the loop of Henle.43 This study and others support the emerging concept of crosstalk between the ADH/vasopressin V2 receptor system (classically considered a regulator of water homeostasis only) and the renin-angiotensin system (classically considered a sodium regulator only), which may modulate renal handling of salt and water further. Urinary concentrating ability is diminished in neonatal kidneys, particularly those of premature infants.14,40 When challenged, term newborns can concentrate urine to an osmolarity of 800 mOsm/kg; preterm infants can concentrate urine to an osmolarity of only 600 mOsm/kg.14 This diminished urinary concentrating ability, particularly in preterm infants, may limit a neonate’s ability to adjust to fluid perturbations—notably perturbations that result in increased free water losses (e.g., increased insensible water losses). Multiple factors limit renal concentrating capacity in preterm infants. Structural immaturity of the renal medulla limits sodium, chloride, and urea movement to the interstitium. Preferential blood flow through the vasa recta limits generation of a medullary gradient. Diminished urea-generated osmotic gradient in the renal medulla limits production and maintenance of the countercurrent mechanisms that are essential in producing maximally concentrated urine. Finally, tubular responsiveness to vasopressin is diminished because of decreased transcription and protein synthesis of AQ2 water channels.69 Urinary dilution capability, in contrast, is normal in term neonates but diminished in preterm neonates. When challenged with a water load, term infants can produce dilute urine with an osmolarity of 50 mOsm/kg, similar to that of an older child or adult. The kidneys of preterm infants, however, may be capable of diluting the urine to an osmolarity of only 70 mOsm/kg.40,55 The diminished urinary diluting and concentrating capacities of neonates have important implications for their care. Excessive fluid restriction places newborns, particularly preterm neonates, at risk for dehydration or hypernatremia or both. Conversely, generous fluid intake poses the risk of intravascular volume overload or hyponatremia or both. High fluid intake has also been associated with an increased risk of symptomatic patent ductus arteriosus (PDA) and necrotizing enterocolitis.10,9 These facts underscore the importance of careful calculation of fluid and electrolyte requirements and close monitoring of fluid balance in high-risk neonates. Calculation of fluid and electrolyte requirements in newborns is based on maintenance needs, deficits, and ongoing losses. Crucial factors that determine these fluid requirements include gestational age, renal function, ambient air temperature and humidity, ventilator dependence, presence of drainage tubes, and gastrointestinal losses.11 Maintenance fluid requirements represent the water required to maintain a newborn in neutral water balance. The total amount of maintenance fluid required is equal to urine production plus insensible losses. Table 44-1 summarizes maintenance fluid requirements during the first month of life for full-term and preterm infants. The numbers presented in Table 44-1 are only guidelines; they are to be used as a starting point for prescribing maintenance fluid for infants with low birth weight during the first week of life. Further adjustments must be based on the clinical situation. In particular, close attention to the patient’s volume status and assessment of factors that may increase or decrease the baseline fluid requirements are essential to the appropriate management of these infants. TABLE 44-1 Maintenance Fluid (Water) Requirements during the First Month of Life Adapted from data from Veille JC. AGA infants in a thermoneutral environment during the first week of life. Clin Perinatol. 1988;15:863; Taeusch W, Ballard RA, eds. Schaffer and Avery’s diseases of the newborn. 6th ed. Philadelphia: Saunders; 1991; and Lorenz J, et al. Phases of fluid and electrolyte homeostasis in the extremely low birth weight infant. Pediatrics. 1995;96:48. Insensible water losses are primarily evaporative losses via the skin and respiratory tract. In newborns, one third of insensible water loss occurs through the respiratory tract, and the remaining two thirds occurs through the skin. Numerous physiologic, environmental, and therapeutic factors can influence insensible water loss, making it the most variable component of the maintenance fluid requirements in newborns. Table 44-2 summarizes the effect of various factors on the degree of insensible water loss in newborns. Transepidermal water loss contributes significantly to increased insensible losses of premature infants (Figure 44-3).20 There is an inverse relationship between body weight and insensible water loss in a neutral thermal environment with moderately high relative humidity.68 Factors that contribute to these increased losses in preterm versus term infants include greater water permeability through a relatively immature epithelial layer of skin, a higher surface area–to–body weight ratio, and increased skin vascularity. Although prenatal glucocorticoids promote maturation of the renal tubules, they do not have a similar effect on skin maturation.26 Infants who have conditions associated with skin breakdown, such as burns or large skin defects such as omphaloceles, also have increased transepidermal water loss. Phototherapy has been reported to increase insensible water losses by up to 26%.38 However, with newer phototherapy techniques, this number may be substantially less.39 TABLE 44-2 Factors Affecting Insensible Water Loss in Newborns Ambient temperature and relative humidity play an important role in influencing transepidermal water loss.7,8,20 An increase in ambient temperature results in increased insensible water loss. A decrease in ambient temperature has no effect on insensible water loss, however, despite the fact that it increases energy expenditure on the basis of cold stress. When ambient temperature is held constant, a lower ambient humidity increases skin water losses because of increased vapor pressure on the skin surface compared with the ambient vapor pressure; this is particularly true for very premature infants. A decrease in humidity from 60% to 20% results in an increased water loss of 100% in infants of less than 26 weeks’ gestation.1 Use of newer humidified incubators has been reported to result in significant decreases in insensible fluids losses and fluid requirements in premature infants.33 Alternatively, in the presence of high relative humidity, water evaporation is less. For example, infants on mechanical ventilation, which provides a humidified oxygen delivery system, have reduced water evaporation from the respiratory tract. Maintenance sodium and chloride should not be provided in the first 1 to 2 days of life because of the relatively volume-expanded state of the newborn. Avoidance of sodium supplementation is particularly important in very premature infants, who have increased water losses and for whom early administration of sodium supplementation is associated with an increased risk of hypernatremia.59 Sodium supplementation from the 4th to the 14th day of life in premature infants was associated with improved developmental outcomes at age 10 to 13 years compared with a group of premature infants who did not receive supplementation.2 Many clinical situations require careful estimates of ongoing pathogenic losses and replacement of deficits. Commonly encountered conditions include diarrhea with dehydration, chest tube drainage, surgical wound drainage, and excessive urinary losses from osmotic diuresis. The important guiding principle in managing patients with these conditions is to measure the volume and composition of the pathogenic losses accurately. Electrolyte losses can be calculated by multiplying the volume of fluid loss by the electrolyte content of the respective body fluids (Table 44-3). TABLE 44-3 Electrolyte Content of Body Fluids During the first few days of life, appropriate fluid and electrolyte balance is reflected by a urine output of approximately 1 to 3 mL/kg per hour, a urine specific gravity of approximately 1.008 to 1.012, and an approximate weight loss of 5% in term infants and 15% in premature infants with very low birth weight.60 Microsampling of serum electrolytes can be done at 8- to 24-hour intervals, depending on illness severity, gestational age, and fluid-electrolyte balance. Extracellular volume depletion is manifest by excessive weight loss, dry oral mucosa, sunken anterior fontanelle, capillary refill greater than 3 seconds, diminished skin turgor, increased heart rate, low blood pressure, elevated blood urea nitrogen, or metabolic acidosis. Serum sodium, which reflects sodium concentration but not sodium content, may be normal, decreased, or increased in states of volume depletion. Bedside monitoring of weight gain, as an indicator of volume status and growth, is essential for monitoring the adequacy of fluid and caloric intake in sick neonates. Beyond the first week of life, infants should gain approximately 20 to 30 g per day. Hyponatremia and/or hypernatremia are extremely common in premature infants as well as in term infants with significant medical issues (such as perinatal asphyxia or septic shock). Hyponatremia, defined as a serum sodium less than 130 mmol/L, occurs in up to 30% of very low birth weight infants in the first week of life and 25% to 65% after the first week.6 Hypernatremia, defined as a serum sodium greater than 150 mmol/L, may occur in up to 40% of preterm infants born at <29 weeks’ gestation.22 Both hyponatremia and hypernatremia have been associated with significant complications. Large changes in serum sodium (either an increase or decrease) in the first month of life in premature infants have been associated with adverse long-term neurologic outcomes.6 However, whether these poor outcomes are caused by the serum sodium changes themselves or, instead, reflect the severity of the infant’s clinical situation, has yet to be established. Hyponatremia in the newborn has historically been delineated as early onset (occurring in the first week of life) or late onset (occurring in the latter half of the first month of life). Given the broad spectrum of gestational ages, underlying diseases, and clinical courses of neonates cared for in modern neonatal intensive care units, these distinctions may be less relevant. Assessing the individual neonate’s clinical status is more important in determining the causes and appropriate interventions for hyponatremia. Increased free water load may be caused by one or more factors, including increased maternal free water intake during labor,27 excess free water administration in the postnatal period, or perinatal nonosmotic release of vasopressin.48 This latter phenomenon may be seen in conditions such as perinatal asphyxia, respiratory distress, bilateral pneumothoraces, and intraventricular hemorrhage47 or with various medications, including morphine, barbiturates, or carbamazepine. Oliguric acute kidney injury or edematous disorders may also contribute to impaired ability to handle a water load. Alternatively, hyponatremia may be due to negative sodium balance. This condition may occur from either inadequate sodium intake or excessive renal losses because of a high fractional excretion of sodium, particularly in preterm infants of less than 28 weeks’ gestation.62 Less common conditions, all of which result in pathologic urinary sodium losses, can cause hyponatremia in neonates and young infants. These conditions may be caused by inherited tubulopathies, such as Bartter syndrome (see Chapter 99), or disorders of aldosterone production or responsiveness. Aldosterone is a steroid hormone produced in the adrenal cortex that has a crucial role in maintaining sodium and potassium homeostasis in the kidney. It is produced in response to either volume depletion, via the renin-angiotensin-aldosterone axis, or an increase in serum potassium. Under the influence of aldosterone, apical epithelial sodium channels are inserted on the luminal (urinary) surface, allowing sodium to be reabsorbed down its concentration gradient. Potassium, as the primary intracellular cation, is excreted in return. This process is facilitated further by aldosterone-mediated increase in the activity of basolateral Na+,K+-ATPase.54 Aldosterone also has a role in acid-base homeostasis and promotes hydrogen secretion (see Acid-Base Management) via actions on H+-ATPase located on the luminal surface of adjacent intercalated cells. Abnormalities in either the production of, or the renal responsiveness to, aldosterone can result in variable degrees of renal sodium wasting, hyperkalemia, and metabolic acidosis. Congenital adrenal hyperplasia is an inherited disorder of cortisol synthesis that results in diminished aldosterone production.63 The most common form is complete absence of 21-hydroxylase activity, a key enzyme in the production of aldosterone. Affected girls have ambiguous genitalia at birth because of excess adrenal androgens. Patients typically present with shock and severe hyponatremia, hyperkalemia, and metabolic acidosis at 1 to 3 weeks of age as the result of a salt-losing crisis. Additional laboratory abnormalities typically seen with this disorder include elevated plasma levels of renin, adrenocorticotropic hormone, 17-hydroxyprogesterone, progesterone, androstenedione, and urinary 17-ketosteroids. Serum cortisol and aldosterone levels may be undetectable. Initial treatment is directed at correcting volume and electrolyte abnormalities. Fluid resuscitation with normal saline should be undertaken immediately, particularly in patients with unstable vital signs and evidence of shock. Hyperkalemia (serum potassium values >7 mEq/L) should be treated initially with insulin and glucose as well as bicarbonate to promote transcellular shifts of potassium into the cells and correct metabolic acidosis. Long-term glucocorticoid and mineralocorticoid replacement therapy should be instituted to facilitate normalization of serum electrolytes and to treat the underlying disease. Sodium supplements may be necessary for a prolonged period. Pseudohypoaldosteronism (PHA) refers to a group of disorders characterized by renal tubular unresponsiveness to aldosterone as evidenced by hyperkalemia, metabolic acidosis, and variable degrees of renal sodium wasting. There are two major subtypes of PHA. Type I usually manifests in infancy with hypotension, severe sodium wasting, and hyperkalemia.49 Type II (Gordon syndrome) typically manifests in late childhood and adulthood, and is not discussed in detail.46 Autosomal recessive type I PHA, previously designated “multiple target organ defects” type I PHA, is a severe, life-threatening systemic disease that affects sodium and potassium handling in the kidney, sweat glands, salivary glands, nasal mucosa, and colon.21 Patients with this disorder usually present in the newborn period with severe salt wasting and life-threatening hyperkalemia. They also have increased sweat chloride that may mimic the presentation of cystic fibrosis. Patients with autosomal recessive type I PHA have a poorer outcome compared with patients with the autosomal dominant form because of the systemic nature of the disease and complete unresponsiveness to mineralocorticoid effects in multiple organs. The disease is caused by mutations in one of three subunits of the amiloride-sensitive epithelial sodium channel of the principal collecting tubule cell, resulting in markedly impaired sodium reabsorption and potassium secretion.49 Sodium chloride supplementation alone often is inadequate in controlling hyperkalemia and metabolic acidosis in these patients. Dietary restriction of potassium intake and the use of rectal sodium polystyrene sulfonate resin (Kayexalate), a sodium-potassium exchange resin, are often required. Indomethacin or hydrochlorothiazide may be necessary to control hyperkalemia and acidosis. These therapies must be continued throughout the child’s lifetime because improvement with age usually does not occur.
Fluid, Electrolytes, and Acid-Base Homeostasis
Fluid and Electrolyte Management
Body Fluid Composition in Fetuses and Newborns
Sodium Balance in Newborns
Water Balance in Newborns
Calculation of Fluid and Electrolyte Requirements
Maintenance Fluids and Electrolytes
Birthweight (g)
Insensible Water Loss (mL/kg/d)
Total Water Requirements by Age (mL/kg/d)
Day 1-2
Day 3-7
Day 8-30
<750
100+
100-200
120-200
120-180
750-1000
60-70
80-150
100-150
120-180
1001-1500
30-65
60-100
80-150
120-180
>1500
15-30
60-80
100-150
120-180
Insensible Losses
Factor
Effect on Insensible Water Loss
Level of maturity
Inversely proportional to birth weight and gestational age (see Figure 44-3)
Environmental temperature above neutral thermal zone
Increased in proportion to increment in temperature
Elevated body temperature
Increased by up to 300% at rectal temperature >37.2°C
High ambient or inspired humidity
Reduced by 30% if ambient or respiratory vapor pressure equals skin or respiratory tract vapor pressure
Skin breakdown (e.g., burn)
Increased; magnitude depends on extent of lesion
Congenital skin defects (e.g., large omphalocele)
Increased; magnitude depends on size of defects
Radiant warmer
Increased by about 50% above values obtained in incubator setting with moderate relative humidity and neutral thermal environment
Phototherapy
Increased by up to 25%, depending on technique
Double-walled incubator or plastic heat shield
Reduced by 10%-30%
Electrolyte Requirements
Pathogenic Losses and Deficit Replacement
Fluid Source
Sodium (mmol/L)
Potassium (mmol/L)
Chloride (mmol/L)
Stomach
20-80
5-20
100-150
Small intestine
100-140
5-15
90-120
Bile
120-140
5-15
90-120
Ileostomy
45-135
3-15
20-120
Diarrheal stool
10-90
10-80
10-110
Fluid and Electrolyte Balance
Hyponatremia and Hypernatremia
Hyponatremia.
Stay updated, free articles. Join our Telegram channel
Full access? Get Clinical Tree
Fluid, Electrolytes, and Acid-Base Homeostasis
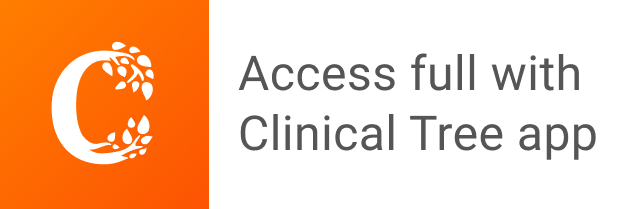