Fluid, Electrolyte, and Acid-Base Disorders
Manjula Gowrishankar
The composition of body fluids, metabolic requirements, and ability to adapt to change differs in infants and children compared to adults. Acute disruptions of fluids and electrolytes or acid-base balance can be life threatening, while chronic titubations interfere with normal growth and development, particularly of the brain and bone.
REGULATION OF BODY FLUID AND ELECTROLYTE DISTRIBUTION
Water is the major constituent of the body. Total body water (TBW) ranges from 60% to 80% of body weight. A newborn infant has close to 80% of body weight as water, while a child over the age of 12 months has 60%. Since adipose tissue has very little water and approximately 80% of muscle tissue is water, a muscular child will have proportionately more water than an obese child. TBW is divided into 2 major compartments: intracellular fluid (ICF), which is 40% of body weight, and extracellular fluid (ECF), which is 20 % of body weight. ECF is further divided into interstitial (16% of body weight) and plasma (4% of body weight) volumes. At birth, ECF volume is approximately 1.5 times that of ICF volume. However, this balance changes rapidly such that ICF volume is equal to ECF volume by day 10 of life, and by 3 years of age, two thirds of TBW is ICF and one third is ECF. Plasma volume is maintained by the oncotic pressure exerted by plasma proteins (albumin). Plasma measurement of electrolytes and acid base is representative of the values for ECF.1
ECF and ICF volume is determined by the content of osmotically active particles within each compartment. Sodium (Na) and its coupled anions (chlorine [Cl] and bicarbonate [HCO3]) are the osmotically active particles that control the ECF volume; potassium (K) and its coupled anions (from macromolecules such as organic phosphates) are the osmotically active particles that control ICF volume. Changes in ECF and ICF volume occur as water moves across the cell membrane through water channels (aquaporins) to achieve osmotic equilibrium. A change in the concentration of sodium in the ECF (plasma) will disrupt the osmotic equilibrium between the ICF and the ECF such that water moves across the cell membrane to achieve a new osmotic equilibrium. Extracellular osmolality is effectively determined by the ECF sodium concentration. This, in turn, determines ICF volume, since total body solute is relatively constant. ICF volume almost always increases (swollen cell) when plasma sodium concentration decreases (hyponatremia), and ICF volume decreases (shrunken cell) when the plasma sodium concentration increases (hypernatremia) (Fig. 466-1).
Regulation of cell volume is extremely important for normal cell function and structural integrity. Acute exposure to a hypotonic ECF causes cells to swell within a few minutes. Shortly thereafter, cell volume begins to decrease toward normal. This decrease is termed a volume regulatory decrease and is due to loss of intracellular solute, particularly potassium. Conversely, exposure to a hypertonic ECF causes cells to decrease in size and then recover via a net leak of solute (mostly Na+ and Cl–) into the cell. However, an increase in these inorganic ions disrupts intracellular enzyme systems. Some cells, such as brain cells, utilize additional mechanisms to control volume with production of substances known as idiogenic osmoles from cellular metabolism. These include taurine, glycine, glutamine, sorbitol, and inositol, which do not disrupt intracellular protein functions. Increases in these idiogenic osmoles have been detected in brain cells as early as 4 hours after an acute hypertonic challenge.
FIGURE 466-1. The circle represents the cell membrane. Normal cell volume is depicted by the light red–shaded area. On the left side of the figure, the cell is placed in a hypotonic environment, which causes water to enter the cell, and the cell swells, as shown by the dark red–shaded area. On the right side of the figure, the cell is placed in a hypertonic environment with resultant water flux out of the cell, and the cell shrinks, as shown by the dark red–shaded area.
Compartment volumes are regulated by integrated mechanisms. These include hypothalamic regulators that control fluid and electrolyte intake and renal water excretion, the renin-angiotensin-adrenal system, and the atrium (arginine vasopressin [AVP] and atrial natriuretic peptide) in response to changes in sodium concentration and extracellular and plasma volumes. These mechanisms and aberrations in function are discussed in more detail in Chapters 525 and 531. In brief, even a small increase in plasma osmolality (1%) is detected by the “osmoreceptor” in the anterior hypothalamus and induces thirst and water intake. It also increases secretion of antidiuretic hormone (ADH), which increases renal water resorption. The converse occurs with decreased plasma osmolality.
The intact kidney responds to diminished renal perfusion such as occurs with decreased plasma (ECF) volume in a predictable and consistent manner. Initially, the glomerular filtration rate is maintained by afferent arteriolar vasodilatation and constriction of the efferent arteriole. Because renal plasma flow is diminished, protein concentration and osmotic pressure are increased in the postglomerular capillaries. This results in enhanced peritubular capillary reabsorption and a nearly maximal reabsorption of sodium in the proximal tubule. Volume contraction also stimulates aldosterone secretion in response to elevated angiotensin II and renin secretion, resulting in maximal sodium reabsorption in the distal tubule and early collecting duct. Together, these forces result in a nearly maximal retention of the filtered load of sodium. Volume depletion also increases the permeability of the collecting ducts; enhanced water reabsorption in this final segment of the nephron results in an increase in urinary osmolality. Thus, when renal perfusion or plasma volume is diminished, the physiological sequence of events that affect both salt and water reabsorption combine to increase intravascular volume.
Atrial natriuretic peptide is produced in the atrium. Secretion is increased by atrial stretch and a variety of other factors induced by hypervolemia. Atrial natriuretic peptide acts in the kidney to increase sodium and water excretion. It also inhibits renin and aldosterone excretion, thus increasing sodium excretion.
MAINTENANCE FLUID AND ELECTROLYTE THERAPY
GENERAL PRINCIPLES
Maintenance fluid requirements were initially established by Holiday and Seeger, who observed that the rate of caloric expenditure is relatively linear for infants and children in 3 weight categories: 100 calories/kg for 1 to 10 kg, 50 calories/kg for 11 to 20 kg, and 20 calories/kg for 21 to 80 kg.2 After 2 endogenous sources of water, water of oxidation and preformed water, are taken into account, fluid requirements are 100 mL of exogenous water for every 100 kcal of energy expended. Thus, a single sliding scale can be used to calculate an estimate of both caloric expenditure and total maintenance fluid volume (see Table 23-4 in Chapter 23). For example, in a child who weighs 14 kg, an estimate of caloric expenditure or maintenance fluid requirement would be 1000 mL for the first 10 kg plus 50 mL/kg for the next 4 kg, or 200 mL, for a total of 1200 mL/d, or similarly, a caloric expenditure of about 1200 kcal/d. By the same approach, the hourly rate of fluid therapy would be 40 mL/h (for the first 10 kg) plus 2 mL/h for next 4 kg, or 48 mL/h. For patients weighing more than 80 kg, the relationship of body weight and water distribution diverges significantly, so this sliding scale calculation is likely to overestimate fluid requirements.
Maintenance fluid is calculated as maintenance fluid = insensible water loss + urine loss. It accounts only for evaporative (insensible) sources and urinary losses under normal physiologic conditions. It does not consider additional fluid lost from the gastrointestinal tract (diarrhea) or alterations in fluid distribution such as the loss of circulating volume into interstitial volume (third spacing), or ascites.
Insensible water loss (IWL) is the solute-free water that evaporates from the lungs to humidify inspired air and from the skin to regulate core body temperature by means of convection and conduction. Skin losses are not ordinary sweat. In the usual environment and under normal physiologic conditions, IWL amounts to about one third of the calculated maintenance fluid (40% in infants and 25% in adolescents) (Table 466-1). Increased IWL should be anticipated when children are hyperthermic or tachypneic, are placed under a radiant warmer, or are exposed to a dry or hot environment. Hyperthermia greater than 38°C increases IWL by 12.5% per 1°C. In contrast, evaporative fluid losses are reduced when children receive humidified air or become hypothermic. The proportion of IWL is about two thirds via skin and one third via lungs.
Table 466-1. Maintenance Water and Electrolytes
Estimated urine fluid losses used for the calculation of maintenance fluid needs is predicated on the ability of the kidney to excrete isosthenuric urine (specific gravity 1.010; urine osmolarity 280–310 mOsm/L) such that urinary losses are assumed to account for 60% to 75% of maintenance volume. For younger children, urine output is anticipated at approximately 2 to 3 mL/kg/h. However, estimation of the volume of urine excreted per day assumes that the kidney is able to concentrate or dilute. If the concentrating ability is impaired (diabetes insipidus, prematurity, sickle cell disease), more water will be excreted; if the concentrating ability is enhanced (increased AVP release for any reason), less water will be excreted. Similarly, if the osmole load is increased (administration of total parenteral nutrition, glucosuria in diabetes mellitus), there will be an increase in the urinary water excretion; if the load is reduced (starvation, low-sodium diet), the urinary water excretion will be reduced. Thus, consideration of factors that may alter urinary output and IWL from the values estimated under normal conditions (maintenance fluid) is imperative in designing appropriate fluid therapy for an ill child.
COMPOSITION OF MAINTENANCE FLUID
The sodium, potassium, and chloride composition in maintenance fluid is based on empiric studies: 3 mmol of sodium and chloride and 2 mmol of potassium are required for each 100 kcal expended, which is extrapolated to the requirement for each 100 mL of fluid lost (Table 466-2). These electrolytes maintain homeostasis and allow for new cell growth in normal children. Thus, the parenteral fluid solution recommended for over 50 years contains 30 mmol/L of sodium and chloride, 20 mmol/L of potassium, and 50 g/L of dextrose (sufficient to avoid ketosis and endogenous protein catabolism).
Table 466-2. Composition of Commonly Administered Intravenous Solutions
Administration of this “maintenance fluid prescription” in children who have undergone fluid losses (dehydration) or with increased levels of ADH (eg, nausea, vomiting, pain, medications, anesthesia, circulating blood volume contraction, meningitis) may be complicated by hyponatremia.3-6 This has led to controversy regarding the optimal electrolyte composition that should be recommended for initial maintenance fluid. Some authors recommend that isotonic saline be administered to all children to avoid hyponatremia, but others are concerned that isotonic saline can produce hypernatremia.7 A reasonable approach is to start therapy with a solution in which the sodium concentration matches plasma sodium until the electrolyte composition and volume of the urine and the estimated IWL are known. In a child with normal serum sodium concentration, this would be isotonic saline. If there is no contraindication (a child with anuria), potassium should be added (20–30 mmol/L) based on the serum potassium concentration. Initiation of this therapy should be followed by serial measurements of the concentrations of urinary sodium and osmolality and urine flow rate as well as serum sodium and potassium. These measures provide information as to whether the child has normal ADH level and activity (normal urine output with specific gravity 1.010 or urine osmolality 280–310 mOsm/L) and the quantity of sodium and potassium lost. Once these data are available, therapy can be designed to match the losses of both water and electrolytes so that the serum sodium and potassium concentrations will remain within the normal range (135–145 meq/L and 3.5–4 meq/L respectively). Generally, a solution with 30 mmol/L sodium and 20 mmol/L potassium given in a volume to match all output (urine and IWL) as per the metabolic demand calculation will maintain normal water, sodium, and potassium levels as long as the kidney is able to either retain or excrete water, sodium, and potassium to maintain homeostasis and there are no other extrarenal losses (cystic fibrosis, diarrhea, vomiting) of water and electrolytes. Determination of a child’s fluid and electrolyte requirement is an ongoing and dynamic process that requires careful monitoring of changes in body weight, serum sodium and potassium, and urine volume (flow rate) and specific gravity or osmolality, urine sodium concentration (UNa), and urine potassium concentration (UK). The composition of commonly administered intravenous fluid is presented in Table 466-3.
DEHYDRATION
Infants and young children are particularly susceptible to dehydration because of their relatively larger body surface area from which fluid is evaporated, a decreased ability to independently access water, and the reduced ability of the kidney to maximally concentrate the urine. Management of the child with dehydration requires (1) estimation of the degree or severity of the dehydration, (2) determination of the type of deficit, and (3) development and implementation of a plan to repair the deficits in both water and electrolytes.
Table 466-3. Severity of Dehydration Based on History and Examination
ESTIMATION OF THE DEGREE AND SEVERITY OF DEHYDRATION
This estimate is based primarily on the patient’s history and physical examination. Laboratory data obtained at the time of initial presentation (eg, plasma urea, albumin, hematocrit) are helpful if premorbid values are available. While clinical observations (Table 466-3) are the cornerstone for estimating the severity of dehydration, they can be misleading. For example, capillary refill is highly susceptible to environmental conditions such as ambient temperature, and heart rate may be increased due to pain or fever. Change in body weight is a very useful parameter to estimate replacement needs. With mild dehydration (3–5%), clinical findings are largely historical—that is, a history of 12 to 24 hours of vomiting/diarrhea, with no change in urine output other than the possibility of a slight decrease in frequency and minimal findings on physical examination. In moderate dehydration (6–10%), there is usually a history of reduced urine output (oliguria), increased thirst (if thirst was intact), and physical findings that include tenting of skin, sunken eyes and fontanel, slight lethargy, and dry lips and mouth. In cases of severe dehydration (11–15%), there are usually additional findings of cardiovascular instability (mottling of skin, tachycardia, hypotension) with neurological changes including extreme irritability or coma.
DETERMINATION OF THE TYPE OF DEFICIT
After determining the degree of dehydration, the next step is to determine the type of dehydration, defined by the tonicity of the patient’s serum (reflected as serum sodium). The commonly used definitions are isotonic (serum sodium 135–145), hypotonic (serum sodium < 135), and hypertonic (serum sodium > 145). Most children with dehydration present with isotonic dehydration where there is both a sodium and a water loss equal to the tonicity of plasma. ECF volume is contracted, but ICF volume remains the same. Thus, the danger to the child is only from ECF volume loss.
In hypotonic dehydration, there is a loss of both sodium and water, but the sodium loss is greater. This may occur if a child with ongoing fluid and electrolyte losses has access to hypotonic fluids (water and juice) such that there is a net gain of water (oral intake, intravenous therapy) without a corresponding gain in sodium.
Hypernatremic dehydration occurs with a hypotonic loss of fluid from the ECF; although there is a loss of both sodium and water, water loss is greater than sodium loss. This may be due to impaired thirst, inability to obtain water, and/or problems with water absorption in the gut or reabsorption in the kidney. Water loss in excess of electrolyte loss may be from water loss from the gastrointestinal tract (as in osmotic diarrhea), skin (heat stroke), or kidney. Incorrect mixing of infant formula may lead to inadequate water intake associated with an obligate urine output required for solute excretion, resulting in negative water balance.
TREATMENT OF DEHYDRATION
All types of dehydration require an assessment of the effective circulating volume. The goals of initial therapy are to prevent or treat shock and improve perfusion to the brain, kidney and gastrointestinal tract by expanding the intravascular volume. The rate and route of fluid administration depend on the severity of dehydration and whether the gut is able to absorb the administered fluid. Mild and moderate dehydration can usually be treated with small, frequent administration of oral rehydration solution (Table 466-4). Some moderate and most severe dehydration require parenteral therapy, at least initially. This is usually given as isotonic saline at volumes of 10 to 30 mL/kg, although some patients may require up to 60 to 100 mL/kg. Ringer lactate can also be used (Table 466-2). However, it contains potassium and lactate (which is metabolized to bicarbonate) and therefore should not be administered in patients with oliguria/anuria or vomiting with resultant metabolic alkalosis. Replacement fluid should be administered rapidly to expand the vascular volume, to prevent or alleviate shock, and to reduce the high levels of ADH present in dehydration.8 This initial therapy is followed by administration of oral or parenteral fluid aimed at replenishing the volume and composition of the body fluids and replacing any ongoing losses.
There are several published regimens designed to guide treatment of a child with dehydration. One convenient online calculation program to assist in intravenous solution formulation is available at http://www.medcalc.com/pedifen.html. However, it is dangerous to follow any particular regimen without a clear understanding of the basic principle, which is to replace what is lost in a 1:1 ratio to maintain homeostasis. Clinically, it may be difficult to determine whether ECF volume has been restored. A reduction in heart rate and establishment of normal urine flow rate (which in a normal young child is approximately 3mL/kg/h) can be utilized as a marker. Frequent measurements of weight, urine output, and serum electrolytes, with appropriate adjustment in administered fluids, is crucial to guide management. In isotonic dehydration, replacement of the deficit can be rapid.9 In contrast, deficit therapy should be slow in both hypotonic and hypertonic dehydration to avoid serious neurologic consequences. As discussed previously, brain cells accommodate to hypertonicity with production of intracellular idiogenic osmoles that maintain cellular size and function. Rapid correction of ECF tonicity results in cellular swelling, with resultant osmotic demyelination syndrome, cerebral edema, and elevations in intracranial pressure.
Accurate calculation of the potassium deficit is impossible because potassium is predominantly an intracellular ion. In addition, depending on factors including the hormonal milieu and metabolic status of the child, the administered potassium may not enter the cells. If renal function is impaired, administered potassium will not be excreted and hyperkalemia can ensue. Therefore, for all types of dehydration, potassium should be administered only after the patient has voided twice and then in concentrations that generally do not exceed 40 mmol/L or a rate of infusion of 0.5 meq/kg/h unless there exists severe hypokalemia.
HYPONATREMIA
Hyponatremia arises from a net loss in sodium or a net gain in water. Any gain of water must be associated with a restriction of the renal excretion of water. Hyponatremia usually follows the ingestion of water or other hypotonic fluids with concurrent loss of sodium. Hypertonic fluid losses due to thiazide diuretics may also cause hyponatremia. The relation between serum sodium, total body sodium, and total body water (TBW) is:
serum sodium = sodium (mmol)/TBW (L)
Measurement of serum sodium, per se, does not reflect serum osmolarity when mannitol or glycine are administered, since they remain in the ECF and cause a shift of water into the ECF that results in hyponatremia but no change in the effective osmolality; although serum sodium is low, there is not a true loss of total body sodium or a true gain in TBW. In contrast, urea and glucose usually do not contribute to effective osmolality because they cross the cell membrane. However, if insulin is absent (diabetes mellitus), glucose acts as an effective osmole and causes water to shift from the ICF to the ECF, resulting in a fall in serum sodium (translocational hyponatremia). Diabetic ketoacidosis with prolonged glucosuria is also associated with a reduction in total body sodium.
Table 466-4. Composition of Commonly Used Oral Rehydration Solutions
Symptoms of hyponatremia result from cellular swelling and dysfunction, especially in the brain, and include headache, lethargy, irritability, muscle cramps, seizures, disorientation, agitation, anorexia, nausea, and even death due to cerebral edema and herniation of the brain. The symptoms and signs depend on the duration and severity of the hyponatremia. Most patients with acute hyponatremia (defined ≤ 48 h in duration) or those with chronic hyponatremia and a further acute decline in serum sodium will be symptomatic.
ASSESSMENT OF HYPONATREMIA
Determination of ECF volume is the first step in identifying the underlying cause of hyponatremia.10 If the ECF volume is reduced, the primary problem is sodium loss. In these cases, measurement of U Na is useful because the normal kidney retains sodium such that the U Na should be less than 10 mmol/L. In such cases, the source of sodium loss is extrarenal (Table 466-5) except in the case of prior vomiting or diuretic use. If the U Naand UK are high, the disorder is one of a sodium-wasting nephropathy (Bartter syndrome), diuretic use, or vomiting. When ECF volume is normal or high, the primary problem is water gain (Table 466-6). Most often, it is due to increased release and/or activity of ADH, whether caused by a low effective circulating volume or other nonosmotic and nonbarometric stimuli. The causes of syndrome of inappropriate ADH (SIADH) are many and include neoplasia, central nervous system and pulmonary disorders, psychosis, pain, nausea, vomiting, and drugs. Water gain may also be due to low water delivery to the distal nephron (renal failure) resulting in decreased water excretion (see Chapter 525).
THERAPY FOR HYPONATREMIA
Hyponatremia requires correction, but the approach and urgency may vary depending on presenting symptoms and chronicity. All patients without a previously documented serum sodium (most patients presenting to the emergency department) should be considered to have chronic hyponatremia. Rapid correction of serum sodium in patients with chronic hyponatremia can result in neurologic consequences, including osmotic demyelination syndrome (previously known as central pontine myelinolysis), hemorrhage, or cerebral edema, and should therefore be avoided. If the patient has acute symptoms such as seizures that are determined to be due to the hyponatremia, it is safe to rapidly raise the serum sodium by 4 to 5 mmol/L.11 This can be achieved by administering 3% sodium chloride (NaCl), which has approximately 513 mmol of sodium in each liter. The volume of 3% NaCl administered is calculated as weight (kg) × 0.6 × 5 × 1.95, where 0.6 adjusts for TBW volume of 60%, 5 is the target increase in mmol/L for serum sodium, and 1.95 is a correction factor (1000 ÷ 513). For example, if a 10-kg child has 60% TBW, the sodium required to raise the serum sodium by 5 mmol/L is 30 mmol (10 × 0.6 × 5). The volume of 3% NaCl to be administered is then calculated to be 58 mL.
Table 466-5. Etiology of Hyponatremia in a Low Extracellular Fluid Volume State
Once the acute symptoms subside, the goal of therapy is to raise the serum sodium by no more than 3 mmol/L during the rest of the 24 hours so that the total correction for the 24 hours is only 8 mmol/L.12 This is equivalent to a rate of correction of 0.3 mmol/L/h. Depending on the etiology of the hyponatremia (primary water gain or sodium loss), either water should be restricted or sodium should be given. In order to accomplish this, all water and electrolyte losses (insensible, urine and others) must be accounted for. Therefore, all losses have to be measured accurately and frequently and replaced or restricted accordingly.
In the example of a 10-kg child, if sodium loss is felt to be the etiology, then a total of 18 mmol of sodium must be given over 24 hours in addition to what was already given and what is being replaced for losses. If water gain is felt to be the cause, and the serum sodium is 120 mmol/L after the initial 3% NaCl administration, then all electrolyte losses have to be replaced 1:1 and the negative water balance calculated as percentage increase in serum sodium desired = (3 mmol/L ÷ 120 mmol/L) × 100 = 2.5%; percentage decrease in TBW should be 2.5% = 6 kg × 2.5% = 150 mL, where 6 kg is the TBW (60% of 10 kg).
In cases of hyponatremia without significant neurologic symptoms, 3% NaCl should not be administered even if the serum sodium is very low. In an asymptomatic patient, the hyponatremia is probably a chronic, long-standing condition. Gradual correction of hyponatremia is safer. Once ECF volume is restored, the stimulus for ADH release can be abolished, resulting in water diuresis. If such a diuresis is not anticipated, the serum sodium may rise much more rapidly than anticipated. Slow correction is preferable with careful monitoring of serum sodium to prevent too rapid a change. Administration of 1-desamino-8d-arginine vasopressin (dDAVP) can be considered in patients with a rapid correction of serum sodium. However, this should be approached with careful consideration. In the case of overcorrection, animal studies and some case reports in adult patients have shown that the development of osmotic demyelination syndrome can be prevented by relowering serum sodium with dDAVP.13
Table 466-6. Etiology of Hyponatremia in Normal or High Extracellular Fluid Volume State
HYPERNATREMIA
Hypernatremia is due to either a gain in total body sodium or a loss in TBW. In general, water loss from the ECF is accompanied by water loss from the ICF and loss of body weight. Exceptions to this relationship are found in cases of prolonged seizure or rhabdomyolysis where cellular swelling occurs because of the deposition of osmotically active particles in the cells without a change in TBW or body weight. Most often, hypernatremia is due to the loss of water. Rarely, true sodium retention occurs as a result of administration of hypertonic NaCl, sodium bicarbonate (NaHCO3), or sodium-rich oral formula and this is usually accompanied by an increase in body weight. Since only a 2% increase in plasma tonicity (a rise in serum sodium from 140 to 143 mmol/L) results in intense thirst, either a defect in thirst mechanisms or a lack of access to water must also be present during the genesis of hypernatremia.
ASSESSMENT OF HYPERNATREMIA
Causes of hypernatremia are listed in Table 466-7. Assessment of body weight as an indicator of ECF volume provides substantial information. If it is increased, sodium gain is likely, and it is if reduced, negative water balance is more likely. Evaluation of urine volume and osmolality also is very helpful. If urine volume is high and osmolality is not maximal, renal losses are the most likely cause. However, if urine volume is low and osmolality is high, then nonrenal causes, including inadequate water intake or other losses, are more likely.
The symptoms and signs of hypernatremia include irritability, lethargy, weakness, seizures, coma, and death. In acute hypernatremia, symptoms develop when the serum sodium exceeds 158 mmol/L. Patients are more symptomatic when there is a rapid rise in the serum sodium. In chronic hypernatremia, higher levels of serum sodium occur with no symptoms being evident. A shift of water out of brain cells with ensuing decrease in brain volume can rupture cerebral veins, resulting in intracerebral and subarachnoid hemorrhages.
Table 466-7. Etiology of Hypernatremia
THERAPY FOR HYPERNATREMIA
As in hyponatremia, cell volume regulatory mechanisms compensate for changes in serum osmolality such that rapid reversal of hypernatremia in chronic hypernatremia can result in osmotic demyelination syndrome, cerebral edema, and increased intracranial pressure. Therefore, correction of serum sodium should not exceed 0.3 mmol/L/h (8 mmol/L/24 h) except in the case of a patient with symptoms such as seizures in which case initial serum sodium reduction by 3 to 4 mmol/L is appropriate. This can be achieved by calculating the water deficit and replacing it. To calculate the water deficit, assume that the rise in serum sodium is due to water loss and that the number of total osmoles in the body have not changed. Free water deficit can be calculated as:
New TBW – Current TBW = [(Current BW × 0.6) × measured serum Na/140] – [current weight × 0.6]
where 140 is the target serum sodium level, and 0.6 is the percentage of TBW. This value is reduced to 0.5 in postpubertal females. The water deficit should be administered at a rate that decreases serum sodium at a rate of 8 mmol/L/24 h. For example, in a patient with a body weight of 10 kg and serum sodium of 170 mmol/L, the water deficit would be calculated as:
10 kg × 0.6 × ([170/140] – 1) = 1.28 L
To decrease serum sodium at a rate of 8 mmol/L/24 h, the calculated water deficit should be administered over about 90 hours: (30 mmol/L ÷ 8 mmol/L/d) = 3.75 days; 3.75 days × 24 h/d = 90h. Therefore the rate of water administration should begin at about 14 mL/h (1280 mL ÷ 90 h). The water deficit can be administered orally or intravenously (dextrose water). During deficit replacement, the patient should be monitored to determine the rate of decline in the serum sodium. In addition to the deficit replacement fluids, all ongoing losses (insensible, urine, and others) have to be replaced in a 1:1 ratio for both water and electrolytes.
POTASSIUM DISORDERS
Potassium is the most abundant intracellular cation (98% of total body content) and plays a fundamental role in cellular homeostasis. Total body potassium is maintained at about 50 mmol/kg body weight with only a small quantity (< 1 mmol/kg) in the ECF. When there is a significant rise or fall in serum potassium, there is a predisposition to cardiac arrhythmias. Therefore, serum potassium is tightly maintained against a concentration gradient that favors movement of potassium from the ICF to the ECF. This is mainly due to a complex system that involves the actions of Na+,K+-ATPase and hormones, including insulin and catecholamines (particularly β-adrenergics; Fig. 466-2).14 A potassium intake (oral or parenteral) must be disposed of immediately to avoid a dangerous increase in the serum potassium; the ICF acts as the sink. However, the extra potassium eventually has to be excreted. The kidneys increase or decrease the rate of excretion of potassium to maintain potassium balance. The intestinal mucosa is also capable of excreting some potassium in chronic hyperkalemia.
The excretion of potassium by the kidney involves 2 mechanisms; one is the active secretion of potassium, primarily by the distal tubule and the collecting duct, and the other is urine flow rate.
Potassium excretion = UK × urine volume
Factors that influence UK are aldosterone activity; presence or absence of non-reabsorbable anions such as bicarbonate, sulfate, or phosphate in the lumen; and sodium delivery. Urine volume is influenced by diuretic agents, urea, sodium, and water delivery.
Evaluation of patients with disorders of potassium requires an evaluation of (1) potassium in-take, (2) shift of potassium into or out of cells, and (3) potassium excretion, which is mainly by the kidneys, although the gastrointestinal tract and skin may also contribute, especially in chronic hyperkalemia. It must be remembered that the serum potassium constitutes a very small portion of total body potassium and that alterations in the serum potassium may not be a reflection of overall total body potassium balance.
HYPOKALEMIA
Hypokalemia is defined as a serum potassium less than 3.5 mmol/L. Most clinical problems related to hypokalemia are due to potassium wasting by the kidneys. As potassium intake decreases, renal potassium excretion decreases markedly to maintain homeostasis. Thus, although a reduced potassium intake can compound an already existing hypokalemia, it is almost never the sole cause of hypokalemia. Causes of hypokalemia include (1) shifts in potassium from the ECF to ICF, (2) excessive non-renal potassium excretion, and (3) increased renal potassium excretion (Table 466-8).
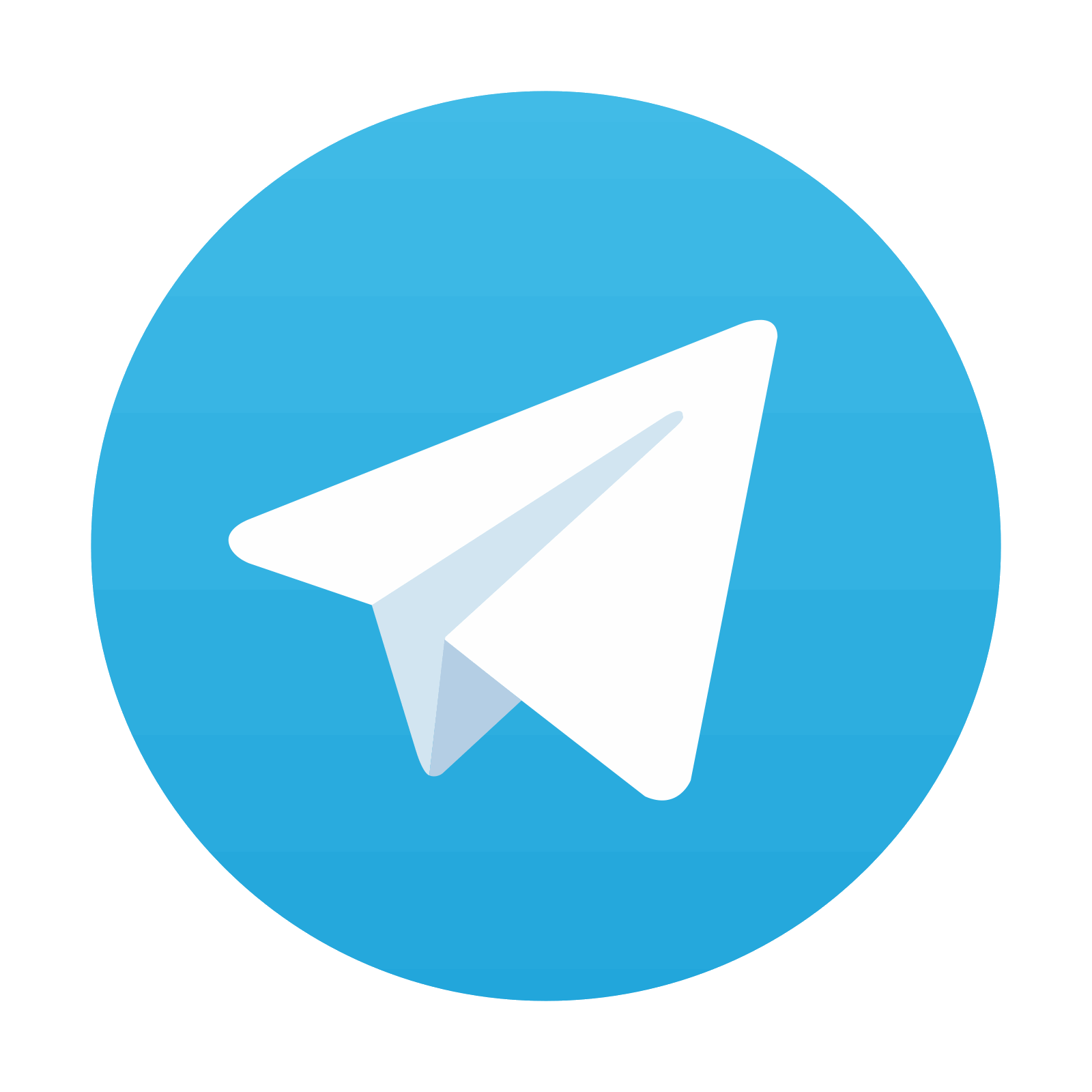
Stay updated, free articles. Join our Telegram channel
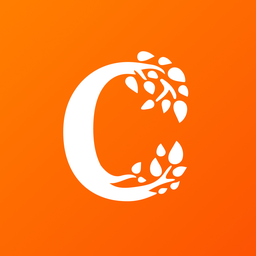
Full access? Get Clinical Tree
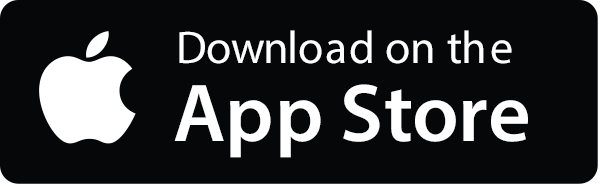
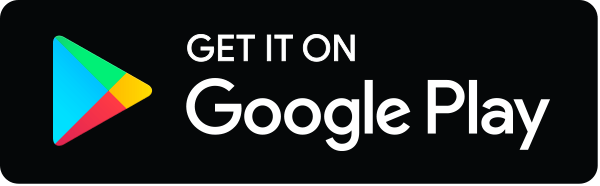