Although the fetus is entirely dependent on the mother for nutrients, it is hormonally independent of the mother (Figure 95-1). Because no maternal peptide hormones are transported to the fetus in any significant amount, fetal endocrine and paracrine responses are mediated by the transport of nutrients such as glucose and amino acids to the fetus. Maternal glucose is the major substrate delivered to the human fetus for energy metabolism.53 Glucose is transported to the fetus along a downward concentration gradient by carrier-mediated transport. In normal pregnancies, the plasma glucose concentration of the fetus is about 70% to 80% of that for the mother. Because maternal-fetal glucose transfer is not saturated by the range of glucose concentrations observed in human pregnancy, even when complicated by diabetes, fetal glucose uptake becomes excessive as the maternal glucose concentration increases. When fetal glucose uptake exceeds the requirements of energy production and growth, the excess glucose is stored as glycogen and triglycerides. The transfer of fatty acids from the mother to the fetus appears to occur only in the free form (i.e., free fatty acids [FFAs]) and to depend on chain length, lipid solubility, and protein binding. A progressive shortening of chain length from C16 to C8 is associated with an increased transfer rate. Protein binding appears to slow the rate of transfer. Contrary to a previously held belief, data suggest that fatty acids are transported in significant amounts from the mother to the fetus.84 A number of proteins involved in the sequestration and transport of fatty acids have been identified in the human placenta.30 In addition, a significant amount of nonessential fatty acid appears to be synthesized de novo by the fetus in utero. Maternal adipose tissue and dietary intake are the primary sources of transplacental long chain polyunsaturated fatty acids (LCPUFA) to the fetus.39,59 β-Hydroxybutyrate and possibly acetoacetate, the major ketone bodies, are transported along a concentration gradient.6 The concentration of β-hydroxybutyrate in fetal blood is significantly less than that in maternal plasma (≈50%); however, a linear correlation between the maternal and fetal concentrations has been reported. Under normal circumstances (i.e., in an uncomplicated, normal pregnancy), the fetus is entirely dependent on the mother for a continuous supply of glucose for both energy metabolism and the synthesis of other metabolic substrates.53 The fetal liver contains the full complement of enzymes required for the synthesis and breakdown of glycogen. Hepatic glycogen content is low early in gestation; a slow, continuous increase occurs between 15 and 20 weeks; and a rapid accumulation of glycogen in the liver is observed late. The fetus also has all the strategic hepatic enzymes involved in gluconeogenesis, although their levels are lower than those in adults.52 The only exception is cytosolic phosphoenolpyruvate carboxykinase, which at least in the rat, is not expressed in utero and appears immediately after birth (Figure 95-2).40 Data in humans in vivo are not available; however, there is a consensus that in the mammalian species studied, the gluconeogenic capacity is not expressed in utero under unperturbed circumstances and the contribution of gluconeogenesis from lactate, pyruvate, or alanine to glucose is quantitatively negligible.64 Studies in humans and animals have consistently demonstrated that the fetus does not produce glucose and that maternal glucose is the only source of fetal glucose. Fluctuations in maternal blood glucose are rapidly reflected in parallel changes in fetal glucose concentration. These variations occur even in response to acute hypoglycemia induced by insulin infusion in the mother during sheep pregnancy. However, if maternal hypoglycemia is prolonged, the fetus begins glucose production.28 Whether such a response can occur in human pregnancy has not been examined and cannot be pursued without violating ethical standards. Glucose is the primary fuel for the fetus, accounting for about 80% of fetal energy consumption. The remaining 20% of fetal energy needs is provided by lactate, amino acids, and other means. The plasma glucose concentration in umbilical vein blood is about 80% of the prevailing maternal blood glucose concentration. After birth, the plasma glucose concentration falls in all infants, reaching its lowest value between 30 and 90 minutes after birth. Thereafter, in full-term healthy neonates, the plasma glucose concentration rises and is maintained at a steady level of 40 to 80 mg/dL. Full-term newborn infants can tolerate fasting without a significant change in the blood glucose concentration. Fasting up to 9 hours after a meal did not cause a decrease in the plasma glucose concentration.28 In full-term infants, the plasma glucose levels obtained at random intervals during the first week after birth have ranged from 40 to 100 mg/dL, with a mean of 80 mg/dL (Figure 95-3).88 The fall in glucose concentrations right after birth appears essential to stimulate physiologic processes that are necessary for postnatal survival, such as promoting glucose production by gluconeogenesis, stimulation of appetite, adaptation to fast/feed cycles, and enhancement of oxidative fat metabolism. These levels are higher than those previously reported in fasting infants and reflect changes in feeding practices and the random nature of measurements. The plasma glucose concentration in healthy, asymptomatic, breastfed babies has been reported to be lower than that in formula-fed infants90 during the first 24 hours of life—an average of 2.1 ± 0.07 mmol/L, or 37 mg/dL (range, 1.2-3.4 mmol/L, or 21-61 mg/dL). Additionally, the lower threshold (<fifth percentile) of plasma glucose levels by population meta-analysis are estimated as 28, 40, and 48 mg/dL in full-term normal newborns at 1 to 2, 3 to 47, and 48 to 72 hours of life, respectively.3 The plasma glucose values in infants who are small for gestational age (SGA) and in premature infants are somewhat lower.43,52 Although no clear definition of hypoglycemia can be described, most investigators consider a plasma glucose level lower than 40 mg/dL to be abnormal, regardless of gestational age (see Chapter 16). The rates of glucose production and use have been measured by a number of investigators with isotopic tracer dilution methods.27 All these studies demonstrated the ability of newborn infants, both full-term and preterm, to produce glucose at rates significantly higher than those in adults. On average, the neonate produces glucose at rates between 4 and 6 mg/kg per minute. The higher rates of glucose production in the neonate reflect the higher ratio of brain to body weight, the brain being the major glucose-using organ. In the first few days after birth, the rate of glucose production during fasting in full-term infants has been reported to decrease slightly. As the infant grows, the rate of glucose production expressed per unit of body weight decreases, so by adolescence, it approaches the rate seen in adults. Hepatic glucose output depends on (1) adequate glycogen stores, (2) sufficient supplies of endogenous gluconeogenic precursors, (3) normally functioning hepatic gluconeogenic and glycogenolytic systems, and (4) a normal endocrine system for modulating these processes. At birth, the neonate has glycogen stores that are greater than those in the adult. However, because of twofold greater basal glucose use, the stores begin to decline within 2 to 3 hours after birth. Muscle and cardiac carbohydrate levels fall more slowly. During asphyxia, the energy requirements are met by anaerobic glycolysis, an inefficient mechanism that results in a limited amount of energy and a decrease in glycogen stores. In premature infants, both total carbohydrate and fat content are reduced, and depletion of liver carbohydrates occurs. Endogenous gluconeogenic substrate availability is probably not a limiting factor because the concentration of plasma amino acids is high at birth as a consequence of active placental transport. Similarly, other gluconeogenic precursors, such as lactate, pyruvate, and glycerol, are also increased. Nevertheless, active gluconeogenesis from alanine and lactate has been demonstrated in the human newborn soon after birth.54,56 Finally, hepatic glucose production is normally regulated; that is, it decreases in response to glucose as well as insulin infusion in both full-term and preterm healthy neonates.49 However, in sick or stressed neonates, exogenous glucose infusion may not completely suppress endogenous glucose production. The transition from an intrauterine environment to independent extrauterine life is also characterized by intense lipid mobilization, as shown by a rapid increase in plasma glycerol and FFAs. In addition, there is a shift in the sources of energy for oxidative metabolism, as evidenced by a decline in the respiratory quotient. An increased contribution of fat to oxidative metabolism is reflected in a drop in the respiratory quotient from near 1.0 at birth to a level between 0.8 and 0.85 within a few hours after birth. Evidence of significant lipolysis in the neonate has been obtained by using isotopic tracers to measure glycerol kinetics.15,74 These studies showed that lipolysis in the neonate occurs at rates almost threefold greater than those in adults and that the major metabolic fate of glycerol released as a result of lipolysis is conversion to glucose. Free fatty acids are used by the heart and muscle in the absence of readily available glucose, producing ketone bodies that readily cross the blood-brain barrier to be used as fuel sources for the brain. Therefore the hypoglycemic neonate may remain asymptomatic because of the ability of utilizing alternate nutrients (ketones and lactate). However, neonatal hypoglycemia caused by hyperinsulinism leads to reduced concentrations of free fatty acids and ketone bodies, depriving the brain of an alternative energy source of ketones for cerebral metabolism. This results in neuroglycopenic symptoms with severe neurologic dysfunction.29 Continuous glucose monitoring using a subcutaneously placed microdialysis sensor has been validated in adults and children with diabetes. The feasibility, safety, and usefulness of these techniques have been examined in babies with low birth weight.11,12 These data show that such devices can be useful for monitoring asymptomatic hypoglycemia, which may be missed by the current practice of intermittent testing. However, these methods cannot measure glucose levels less than 45 mg/dL with confidence, and the variance is large at high glucose concentrations. Thus far, these techniques have been used only for research studies, and their associated potential risks require further evaluation. Perturbations in glucose metabolism after birth caused by failure to adapt to the extrauterine environment, as a result of either alterations in maternal metabolism or intrinsic metabolic problems in the neonate, often result in hypoglycemia. Lengthy debate has occurred among investigators regarding the definition of hypoglycemia.1,22,82 The controversy arises partly because of the spontaneous decrease followed by recovery of the blood glucose concentration after birth and partly because many neonates have very low blood glucose concentrations without any clinical signs or symptoms (i.e., asymptomatic hypoglycemia). Finally, a convincing relationship between asymptomatic hypoglycemia in the neonate and long-term neurologic sequelae has not been demonstrated. Further, preventing and treating neonatal hypoglycemia remains largely empiric.1 Attempts have been made to define hypoglycemia by either a statistical approach or correlation of blood glucose concentration with clinical signs and symptoms. The presence or absence of such signs cannot be used reliably to define neonatal hypoglycemia owing to immaturity of counter-regulatory hormonal stress response responsible for classic symptoms of hypoglycemia in older children and adults. Because the clinical signs of hypoglycemia in the neonate are not specific to alterations in glucose concentration, this approach has not been successful. The statistical definition of hypoglycemia is based on surveys of large numbers of infants. Abnormality is defined as a blood glucose concentration that falls outside a prescribed limit, for example, outside two standard deviations from the norm.22,62 However, since that time, the intrapartum clinical care of the mother and nutritional clinical management of the neonate have changed markedly, so the glucose concentrations observed several years ago are no longer seen in current clinical practice. In addition, the routine use of intravenous dextrose-containing fluids in preterm infants has confounded the ability to study glucose concentration in these small babies. Using 95% confidence intervals of the mean, Srinivasan and colleagues88 showed that normal, healthy, full-term infants who were fed early achieved plasma glucose values of higher than 40 mg/dL within 4 hours after birth and higher than 45 mg/dL within 24 hours after birth. In their study, 10% of the neonates had at least one value of less than 35 mg/dL in the first 3 hours (see Figure 95-3). A cutoff value (although arbitrary) of 40 mg/dL has been developed by the American Academy of Pediatrics for the treatment with intravenous glucose of infants with symptomatic hypoglycemia, a value that is higher than the physiologic nadir and higher than concentrations usually associated with clinical signs.1 These new AAP guidelines also recommend screening in the first 24 hours of life of all asymptomatic at-risk infants for neonatal hypoglycemia (preterm infants at 34 weeks to 36 6/7 weeks and term infants who were born to mothers with diabetes, small for gestational age, or large for gestational age).1 The clinical manifestations of hypoglycemia are nonspecific and similar to those of many disorders in newborn infants (Box 95-1). The clinical signs and symptoms of hypoglycemia should improve with correction of the low glucose concentration. In addition, careful attention should be given to ensure that other associated disorders (e.g., sepsis, asphyxia) are not missed. Because of its implications for both long-term prognosis and clinical management, it is worthwhile to consider two types of neonatal hypoglycemia: those cases limited to the newborn period (transient hypoglycemia) and those cases continuing over an extended period of time or occurring more than once (persistent or recurrent hypoglycemia) (Box 95-2). Transient hypoglycemia is often a consequence of changes in the metabolic environment in utero or ex utero, whereas persistent or recurrent hypoglycemia arises from intrinsic metabolic problems in the infant. Either type of hypoglycemia may manifest asymptomatically or symptomatically. As discussed, the fetus is entirely dependent on the mother for the supply of glucose, and fetal glucose concentration closely mimics that of the mother.6 An increase in maternal glucose concentration as a result of exogenous glucose infusion causes an increase in fetal glucose concentration, which in turn causes an increase in fetal insulin levels. Studies in animals have shown that fetal hyperinsulinism, caused by either direct infusion of insulin to the fetus or fetal hyperglycemia, results in an increased metabolic rate in the fetus, fetal hypoxemia, and metabolic acidosis. Similar patterns of change have been demonstrated in human pregnancy.77 The acute administration of glucose during the intrapartum period—for example, to prevent hypotension during the conduction of anesthesia—has been shown to result in increased glucose, insulin, and lactate levels in the cord blood obtained at delivery.56,77 Fetal hyperinsulinism leads to hyperinsulinemia and hypoglycemia in the neonate. Similar observations have been reported in the diabetic pregnancy. Therefore, caution should be exercised in the administration of glucose to the mother during labor and delivery (see Chapter 28). Blood glucose concentrations in the mother should not be allowed to exceed those observed in the normal physiologic range. Oral beta-sympathomimetic tocolytic drugs such as terbutaline and ritodrine have caused sustained hypoglycemia and elevated cord blood insulin levels in infants delivered within 2 days after termination of tocolytic therapy (see Chapter 19). These agents may cause neonatal hypoglycemia through maternal hyperglycemia and fetal hyperglycemia and hyperinsulinemia. Although perturbations in glucose metabolism in the mother and their consequences to the fetus and neonate constitute the major metabolic disturbances of diabetes in pregnancy, neonatal morbidity involves a number of organ systems.48 (see Chapter 19) The major neonatal morbidities in infants of diabetic mothers (IDMs) whose maternal diabetes was not rigorously managed are displayed in Box 95-3. The pathogenesis of the spectrum of fetal and neonatal morbidities in the IDM has now been described by a number of careful studies in human and animal models. Intermittent hyperglycemia in the mother results in hyperglycemia in the fetus, which in turn causes hypertrophy of the fetal pancreatic islets and beta cells and increased secretion of insulin. Because of the lack of significant transfer of insulin from the mother to the fetus in humans, the circulating insulin in the fetal compartment is mostly of fetal origin. However, two studies have demonstrated that in insulin-dependent diabetes, in the presence of antibodies to insulin, a small amount of insulin exogenously administered to the mother may be transported to the fetus.10,65 Nevertheless, the newborn IDM has been shown to be hyperinsulinemic, as evidenced by increased levels of insulin and C peptide in the umbilical blood. The consequences of fetal hyperinsulinemia have been documented in experimental animal models. Direct insulin infusion into the normal rhesus monkey fetus induces fetal hyperinsulinemia, resulting in macrosomia, cardiomegaly, and an increase in adipose tissue. In addition, the activity of hepatic enzymes affecting lipid synthesis increased. Chronic fetal hyperinsulinemia also results in an increase in the metabolic rate and oxygen consumption, leading to relative hypoxemia, which in turn results in an increase in the synthesis of erythropoietin and an increase in red blood cell mass and polycythemia. In addition, hyperinsulinemia has been shown to suppress the production of surfactant in the lung and thus predispose to respiratory distress syndrome after birth (see Chapter 70). An excessive accumulation of glycogen in the liver, adipose tissue, and other tissues has also been observed in IDMs. The sequence of these metabolic events is outlined in Figure 95-4. After birth, the newborn IDM whose mother’s disease has not received rigorous antepartum management appears to be unable to produce sufficient circulating glucose and alternative fuels. Even infants of mothers with rigorous management (i.e., those who have maintained normoglycemia throughout gestation) develop significantly lower blood glucose concentrations than those of normal infants. In addition, they do not mobilize fatty acids from adipose tissue; as a result, their circulating levels of FFAs remain low, although a normal increase in plasma glycerol has been observed. Such a metabolic picture suggests a persistent insulin action and the lack of a counter-regulatory hormonal response. The latter is confirmed by the lack of an increase in circulating glucagon and catecholamine levels in IDMs during hypoglycemia. The combination of hyperinsulinism and insufficient counter-regulation results in decreased hepatic glucose production, increased peripheral glucose uptake, and impaired lipolysis. When measured by the isotope tracer dilution method, IDMs produce glucose at significantly lower rates than normal infants.55 In addition, their basal metabolic rates, or rates of oxygen consumption, have been reported to be lower than those of normal infants. It should be recognized that the clinical manifestations described here relate to diabetic mothers whose metabolism has not been well controlled. Data from a study7 in which maternal diabetes was rigorously managed by either an insulin infusion pump or split-dose insulin therapy are shown in Table 95-1. Despite rigorous management and reduced maternal hyperglycemia, significant morbidity may persist. TABLE 95-1 Neonatal Outcome in Infants of Rigorously Managed Diabetic Mothers *The K score is a method of adjusting the birth weight in grams for the infant’s gestational age. A K score of 1 represents the 90th percentile for weight; scores of 0 and −1 represent the 50th and 10th percentiles, respectively. Data from Aucott SW, et al. Rigorous management of insulin-dependent diabetes mellitus during pregnancy. Acta Diabetol. 1994;31:126. At birth, these infants are obese, plethoric, and large for gestational age (LGA) and show evidence of excessive fat as well as visceromegaly in the form of a large liver, spleen, and heart (Figure 95-5).48 Because the growth of the brain and possibly the kidney is not dependent on insulin, these two organs are normal in size. Careful management of maternal metabolism tends to reduce the incidence of macrosomia but does not prevent it. The incidence of congenital malformations is increased twofold to threefold in the infants of insulin-dependent diabetic mothers compared with the normal population. The frequency of congenital anomalies is not increased in the infants of gestationally diabetic mothers or in those of diabetic fathers. A developmental morphologic approach, as applied by Mills and colleagues, suggests that congenital malformations in IDMs occur before the seventh week of development.68 A number of congenital anomalies, including those of the heart, musculoskeletal system, and genitourinary system, have been reported. Caudal agenesis or dysplasia syndrome (Figure 95-6) is seen with markedly increased frequency, especially in IDMs. This syndrome consists of agenesis or hypoplasia of the femora in conjunction with agenesis of the lower vertebrae and sacrum. Data from animal models show that certain genes belonging to the PAX family are involved in enhancing apoptosis, which then results in structural defects, for example, neural tube defects. The frequency of congenital anomalies is significantly increased in mothers with poor metabolic control, as evidenced by increased hemoglobin A1c levels early in gestation,67 and a significant decrease in congenital malformations has been reported with rigorous metabolic regulation in the periconceptional period (see Chapter 19).35 See Chapter 96. Alterations in calcium and magnesium homeostasis occur in about 50% of infants born to insulin-dependent diabetic mothers. Unlike hypoglycemia, hypocalcemia becomes apparent between 48 and 72 hours after birth. Plasma calcium concentrations of lower than 7 mg/dL are frequently observed. Hypocalcemia has been related to the severity and duration of maternal diabetes. In addition, it may be potentiated by prematurity and asphyxia. The mechanisms of hypocalcemia are probable failure of the IDM to mount an appropriate parathyroid hormone (PTH) response, persistently high levels of calcitonin, and possible alterations in vitamin D metabolism. Hypomagnesemia (≈1.5 mg/dL) has also been frequently observed. It is usually transient, and its pathophysiologic significance remains uncertain. The management strategies for other clinical problems, such as polycythemia (see Chapters 88 and 89), hyperbilirubinemia (see Chapter 100), and renal vein thrombosis (see Chapter 101), that occur with slightly higher frequency in IDMs are similar to those in otherwise normal infants. Septal hypertrophy and cardiomegaly are specific phenotypic consequences of diabetes in pregnancy and may be manifested with heart failure in the neonate (Figure 95-7; also see Chapter 81). In addition, data in asymptomatic infants of gestationally diabetic mothers have shown alterations in diastolic function and decreased passive compliance of the ventricular myocardium. By serially evaluating cardiac growth in utero in the fetuses of diabetic mothers, it has been shown that despite good metabolic control, cardiac hypertrophy developed in late gestation (34-40 weeks). Although IDMs with septal hypertrophy may present with obstructive left heart failure, they may also be asymptomatic. Follow-up data show that cardiomyopathy in an IDM is a transient disease and usually disappears spontaneously within 6 months after birth. Otherwise minor functional changes, such as impaired diastolic filling, have been reported in infants of gestational diabetic mothers and are usually not clinically significant. See Chapter 19. Strict metabolic control, improved fetal surveillance, early delivery, and neonatal intensive care have led to improved survival among IDMs. Preconceptional and early postconceptional metabolic control may decrease the incidence of congenital malformations. Improving maternal compliance, preventing ketoacidosis, and recognizing and treating pregnancy-induced hypertension and pyelonephritis are particularly important. Attempts should be made to maintain maternal fasting plasma glucose values at less than 80 mg/dL and 2-hour postprandial plasma glucose values at less than 120 mg/dL. With appropriate ambulatory support, hospitalization is not required in most mothers. Indications for hospitalization include pregnancy-induced hypertension, acute or chronic polyhydramnios, infections, and poor metabolic control. Early screening for congenital malformations includes a determination of the maternal serum alpha-fetoprotein level for open neural tube defects and a level II ultrasound at 18 to 20 weeks of gestation (see Chapters 11 and 12). Follow-up ultrasonography is useful in the evaluation of amniotic fluid volume, fetal growth, and placental grading. Tests of fetal well-being begin in the second trimester (28-32 weeks of gestation). These tests usually include daily fetal movement counts and biweekly biophysical testing (non-stress testing, biophysical profile, or both) or non-stress testing combined with an amniotic fluid index (see Chapter 13). Delivery in insulin-dependent women is indicated after documentation of fetal lung maturity by amniocentesis (see Chapter 70). With appropriate care and fetal surveillance, most patients are now monitored expectantly for the spontaneous onset of labor at term. Vaginal delivery is preferred, but obstetric factors may justify cesarean delivery. Caution should be exercised in the delivery of a macrosomic fetus at risk for traumatic complications. During labor, glucose and insulin therapy should be adjusted to maintain normoglycemia in the mother. Even though physiologic and clinical data clearly demonstrate a marked reduction in fetal and neonatal morbidity and mortality in pregnancy with diabetes, studies from the United Kingdom failed to show that such a goal was achieved in clinical practice.19,47,86 Continuous rigorous surveillance of diabetes throughout the pregnancy and fastidious management of altered metabolism are required.
Disorders of Carbohydrate Metabolism in the Neonate
Placental Transport of Nutrients: Maternal-Fetal Relationship
Glucose
Fatty Acids
Other Fetal Substrates
Fetal Glucose Metabolism
Glucose Metabolism After Birth
Measurement of Glucose
Hypoglycemia
Transient Hypoglycemia Caused by Changes in Maternal Metabolism
Intrapartum Glucose Administration
Maternal Pharmacologic Treatment
Diabetes in Pregnancy: The Infant of a Diabetic Mother
Clinical Manifestations.
Parameter
Infants of Diabetic Mother (N = 78)
Controls (N = 78)
P
Birth weight (g)
3454 ± 817
3271 ± 621
—
Gestational age (wk)
37.9 ± 0.95
39.3 ± 1.7
.0001
K score*
0.77 ± 0.95
0.18 ± 0.91
.0001
Macrosomia (>4 kg)
19 (24%)
8 (10%)
.03
Large for gestational age
32 (41%)
12 (15%)
.0002
Hypoglycemia
11 (14%)
1 (1%)
0.0025
Hyperbilirubinemia
36 (46%)
18 (23%)
.002
Respiratory distress syndrome
9 (12%)
1 (1%)
.008
Admission to neonatal intensive care unit
21 (27%)
9 (12%)
.01
Macrosomia.
Congenital Anomalies.
Hypocalcemia and Hypomagnesemia.
Septal Hypertrophy of the Heart.
Management of the Diabetic Mother.
Stay updated, free articles. Join our Telegram channel
Full access? Get Clinical Tree
Disorders of Carbohydrate Metabolism in the Neonate
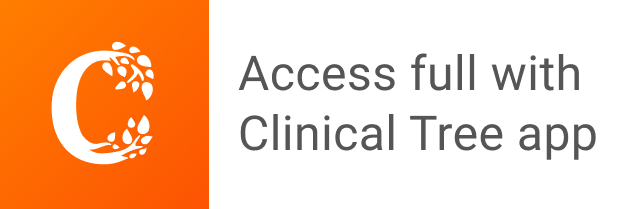