Disorders of Calcium, Phosphorus, and Magnesium Metabolism in the Neonate
Approximately 98% of the calcium, 80% of the phosphorus, and 65% of the magnesium in the body are in the skeleton; these elements, often referred to as the “bone minerals” are also constituents of the intracellular and extracellular spaces. The metabolism of these bone minerals and mineralization of the skeleton are complex functions that require the interaction of various parameters. These include an adequate supply of nutrients, including proteins for collagen matrix synthesis, and an adequate intake and absorption of calcium and phosphorus for full bone mineralization.75 During prenatal development, nutrients are transferred mainly across the placenta. From the analysis of stillbirths and deceased neonates, it has been calculated that during the last trimester of gestation, the daily accretion per kilogram of body weight represents approximately 100 to 130 mg of calcium, 60 to 70 mg of phosphorus, and 3 mg of magnesium.86 Therefore, at birth, the whole-body content of a term infant represents about 30 g of calcium, 16 g of phosphorus, and 0.75 g of magnesium. After birth, nutrient intake from most enteral sources, especially unfortified human milk, is below the amount needed to achieve this level of mineral retention.81 This can promote the occurrence of relative osteopenia or clinical rickets in preterm infants and in some high-risk full-term infants.3 In addition to their role in bone formation, the bone minerals play important roles in many physiologic processes such as transport across membranes, activation and inhibition of enzymes, intracellular regulation of metabolic pathways, secretion and action of hormones, blood coagulation, muscle contractility, and nerve conduction. The 20% of phosphorus that is not complexed within bone is present mainly as adenosine triphosphate, nucleic acids, and cell and organelle membranes. Magnesium, an essential intracellular cation, is critical in energy-requiring metabolic processes, protein synthesis, membrane integrity, nervous tissue conduction, neuromuscular excitability, muscle contractility, hormone secretion, and intermediary metabolism.
Calcium, Phosphorus, and Magnesium Physiology
Calcium Physiology
Serum Calcium
Serum calcium represents a very small fraction of total-body calcium. Less than 1% of whole body calcium is present in extracellular fluid and soft tissues. In the circulation, calcium is distributed among three interconvertible fractions. About 50% of total serum calcium is in the ionized form at the normal serum protein concentration and represents the biologically active component of the total serum calcium concentration. Another 8% to 10% is complexed to organic and inorganic acids (e.g., citrate, lactate, bicarbonate, sulfate, and phosphate). Together, the ionized and complexed calcium fractions represent the diffusible portion of circulating calcium. About 40% of serum calcium is protein bound, primarily to albumin (80%), but also to globulins (20%).41 Ionized calcium is the only physiologically active fraction. The protein-bound calcium is not biologically active but provides a rapidly available reserve of calcium. Under normal circumstances, the serum calcium concentration is tightly regulated by parathyroid hormone (PTH) and calcitriol (1,25-dihydroxy vitamin D3; 1,25[OH]2D3), which increase serum calcium (Figure 96-1), and by calcitonin, which decreases serum calcium.

Serum total and ionized calcium concentrations are relatively high at birth but decrease sharply during the first hours of life to reach a nadir at 24 hours and increase progressively thereafter up to the end of the first week of life (Table 96-1). Sudden changes in the distribution of calcium between ionized and bound fractions may cause symptoms of hypocalcemia even in children with functioning hormonal mechanisms for the regulation of the ionized calcium concentration. Increases in the extracellular fluid concentration of anions such as phosphate, citrate, or bicarbonate increase the proportion of bound calcium and decrease ionized calcium. Alkalosis increases the affinity of albumin for calcium and thereby decreases the concentration of ionized calcium. In contrast, acidosis increases the ionized calcium concentration by decreasing the binding of calcium to albumin. Although it remains common to measure the total serum calcium concentration, more physiologically relevant information is obtained by direct measurement of the ionized calcium concentration. The total serum calcium decreases about 0.8 mg/dL, or 0.25 mmol/L, for each 1 g/dL of decrease in serum albumin, without any change in the ionized calcium.
TABLE 96-1
Early Life Changes in Serum Calcium, PTH, and Vitamin D Levels in Full-Term and Preterm Infants
Cord Blood | 24 hr | 48 hr | 96-120 hr | 30 Days | |
Calcium, nmol/L | |||||
Full-term | 2.42 ± 0.08 | 2.17 ± 0.10 | 2.16 ± 0.08 | 2.22 ± 0.12 | 2.52 ± 0.08 |
Preterm | 2.28 ± 0.09 | 1.91 ± 0.06 | 1.86 ± 0.07 | 2.08 ± 0.11 | 2.43 ± 0.06 |
Intact PTH, pg/mL | |||||
Full-term | 5.1 ± 3 | 33 ± 8 | 30 ± 5 | 28 ± 16 | |
Preterm | 4.5 ± 3 | 72 ± 17 | 56 ± 20 | 36 ± 14 | |
25(OH)D, ng/mL | |||||
Full-term | 13 ± 3 | 12 ± 2 | 12 ± 2 | 17 ± 1 | |
Preterm | 10 ± 3 | 8 ± 2 | 12 ± 2 | 17 ± 2 | |
1,25(OH)2D, pg/mL | |||||
Full-term | 38 ± 4 | 74 ± 9 | 100 ± 5 | 61 ± 4 | |
Preterm | 37 ± 6 | 62 ± 9 | 128 ± 29 | 108 ± 13 |
Data from Hochberg Z, ed. Vitamin D and rickets. Endocrine development, vol 6. Basel, Switzerland: Karger; 2003:34–49.
Placental Transport
During pregnancy, calcium is actively transferred from the mother to the fetus. The normal fetus accumulates about 25 to 30 g of calcium by the end of gestation. Approximately 80% of this calcium accumulates during the third trimester, when the fetal skeleton is rapidly mineralized. To meet the high demand for mineral requirements of the developing skeleton, the fetus maintains higher serum calcium and phosphorus levels than the maternal levels. This process is the result of the active transport of calcium across the placenta by a calcium pump in the basal membrane that maintains a gradient of maternal-to-fetal calcium of 1 : 1.4.
The main regulator of fetal ionized calcium appears to be parathormone-releasing protein (PTHrP), which is produced by the placenta as well as by the fetal parathyroid glands. Animal data suggest that both PTH and PTHrP act in the regulation of fetal mineral metabolism. PTH-ablated mice showed significant abnormalities in fetal bone formation, including decreased mineralization of the cartilage matrix and decreased vascular invasion of the growth plate, suggesting a role of PTH beyond simply regulating serum mineral ion concentration.60 In contrast to the relatively mild phenotype of PTH ablated mice, animals that lack PTHrP show major developmental abnormalities, particularly a significant acceleration of chondrocyte differentiation in the growth plates leading to a lethal skeletal dysplasia characterized by the premature mineralization of all bones that are formed through an endochondral process.46,51
Although vitamin D is critical for mineral ion homeostasis and bone development during adult life, fetal mineral ion homeostasis appears to be mostly independent of this hormone. 1,25(OH)2D3 is produced by the fetal kidney, and the vitamin D receptor is present in many fetal tissues, including the placenta. However, neonates deficient in 1-alpha-hydroxylase are grossly normal from birth until weaning.28 Additionally, the absence of the vitamin D receptor (VDR) either in the mother or the fetus does not affect placental calcium transfer or fetal serum levels of calcium, phosphorus, or magnesium during the first days after delivery, indicating that the VDR in either side of the placenta, on the maternal or at the fetal side, is not critical for placental calcium, phosphorus, or magnesium transfer.52,89 Cord concentrations of 25(OH)D and 1,25(OH)2D3 correlate significantly with those found in the maternal circulation, suggesting that the vitamin D pool of the fetus depends entirely on that of the mother. 1,25(OH)2D3 found in fetal plasma is a result of fetal kidney and placental hydroxylation activity. The placenta is able to synthesize and metabolize 1,25(OH)2D3 through the activity of 25-hydroxyvitamin D-1α hydroxylase and 1,25-dihydroxyvitamin D-24 hydroxylase, two key enzymes for vitamin D metabolism. Bone mass of neonates may be partially related to the vitamin D status of the mother, although this relationship is not consistently found in either the United States or in developing countries.79
After the umbilical cord has been cut, the neonate loses the transplacental supply of calcium and must adapt rapidly to ensure normal calcium homeostasis and skeletal growth and mineralization. The neonate is dependent on intestinal absorption to supply calcium and therefore must quickly stimulate the synthesis of PTH and 1,25(OH)2D3, which had been suppressed in utero. Total and ionized calcium levels decrease significantly within 24 to 48 hours after birth. Calcium concentrations usually return to normal by 5 to 8 days after birth. Serum PTH levels, which are low at birth, increase within the first 24 to 48 hours, probably in response to the decrease in serum calcium. Subsequently, 1,25(OH)2D3 levels rise to adult levels within a few days. Serum calcitonin rises two- to tenfold over cord blood levels within the first 48 hours. Hypocalcemic premature and asphyxiated newborns have the highest levels of postnatal calcitonin.64
Intestinal Absorption
Calcium absorption occurs primarily in the small intestine by both active and passive processes. Ionization of calcium compounds, which requires an acidic pH, develops in the stomach and is a prerequisite for absorption. Vitamin D is essential for the active absorption of calcium, which involves carriers such as calcium-binding proteins. However, the role of vitamin D and active transport early in life is uncertain.
The absorption rate of calcium is higher than that during other periods of life except for during pregnancy in populations with low mineral intake. The average reported absorption of calcium from human milk in small infants is approximately 50% to 60% of its intake. The absorption of calcium added to human milk with commercially available fortifiers generally parallels that of the calcium endogenous to human milk.36
Studies in vitamin D receptor-knockout (VDR-KO) mice have underscored the importance of an intact VDR for intestinal calcium absorption. The VDR-KO mice exhibit decreased calcium absorption with a concomitant reduction in the expression of transcellular calcium transport proteins. Vitamin D-dependent transcellular calcium transport involves three major components: transient receptor potential vanilloid type 6 (TRPV6), calbindin D9k, and Ca+2ATPase. VDR-KO mice exhibit decreased calcium absorption with a concomitant reduction in the expression of transcellular calcium transport proteins. However, recent studies revealed that TRPV6/calbindin D9k double KO mice do not demonstrate impaired intestinal calcium transport when they have usual dietary calcium intake, indicating that other compensatory factors are involved in intestinal calcium transport. Several studies underscored the importance of the paracellular route and suggested that 1,25(OH)2D3 regulates tight junction proteins such as Caludin-2,3, Caludin-12, and Cadherin-17, which lead to increased paracellular calcium transport.25
Passive calcium transport is driven by chemical gradients that represent movement of calcium among the cells (paracellular transport). It may account for most of the calcium absorption very early in life, particularly in premature infants in whom the transport, which is transcellular and dependent on vitamin D, is not completely expressed.18,19 In addition to vitamin D status, various other factors affect calcium absorption. Ionization of calcium compounds, which requires an acidic pH, occurs in the stomach and is a prerequisite for absorption. Therefore, low availability could be the result of an insoluble fraction of calcium intake or the precipitation of calcium in the gut. The quantity and quality of fat intake may influence calcium absorption through the formation of calcium soaps. It has been suggested that the free palmitate content in the gastrointestinal tract after the hydrolysis of triglyceride may impair calcium absorption.20 Human milk contains a bile salt-stimulated lipase that is not specific to the Sn-1 and Sn-3 positions. The improvement of calcium absorption with the use of medium-chain triglycerides may be the result of the reduction of total saturated long-chain fatty acid content in formula. Relatively low gastrointestinal pH content or the lactose and casein content of the formula may also have an additional positive effect on calcium absorption.
In infants, a significant amount of calcium is secreted in the intestinal lumen through digestive fluid. With the use of stable isotopes, endogenous fecal calcium excretion and true calcium absorption may be evaluated. Numerous metabolic balance studies have been performed in preterm infants fed human milk or a formula to evaluate apparent calcium absorption. In preterm infants fed human milk with or without fortification, calcium absorption ranges from 50% to 70%. In formula-fed infants, the percentage of calcium absorption may be slightly less than that with human milk although the difference does not appear to be large.3
Renal Excretion
Under normal circumstances, calcium status is maintained by a balance between its intestinal absorption and renal and endogenous fecal excretion with a small component of skin losses, especially with sweating. Although it is responsible for only 5% to 10% of Ca2+ reabsorption, the major regulation of Ca2+ reabsorption occurs in the distal convoluted tubule by a mechanism independent of Na+ reabsorption, but regulated by PTH and 1,25(OH)2D3. Calcium reabsorption is increased by both PTH and 1,25(OH)2D3. It is also regulated by ionized calcium concentration, phosphate concentration, and acid-base status. It increases with Ca2+ depletion and alkalosis but decreases with hypercalcemia, phosphate depletion, and acidosis. The effects of diuretics on renal calcium excretion vary considerably. Furosemide markedly increases renal calcium losses and is a risk factor for neonatal nephrocalcinosis. Thiazides increase renal tubular calcium reabsorption, thereby reducing calciuria. However, the clinical consequences of this process on neonatal physiology have not been fully investigated.84
Nearly all filtered calcium (98%) is reabsorbed in the renal tubule. However, preterm and term infants differ from adults in three main aspects: (1) renal function is far from being completely developed; (2) mineral requirements for growth are very high; (3) renal calcium load results solely in the difference between net absorption and net bone and soft tissue retention. Considering that calcium soft tissue retention is negligible, renal calcium load is highly dependent on bone calcium deposition associated with phosphorus in the form of hydroxyapatite [Ca10(PO4)6(OH)2] containing a molar calcium-to-phosphorus ratio of 1.67 (2.15 wt/wt). Therefore, the main determinant of the urinary loss of calcium in preterm and term neonates is phosphorus depletion and urinary excretion. When human milk is provided exclusively, there is an increase in the urinary excretion of calcium associated with very low urinary phosphate excretion.84 In contrast, when human milk is supplemented with phosphate, significant phosphaturia appears at the same time that calciuria decreases to a minimum level. Therefore, hypercalciuria can primarily be explained by a relative phosphate depletion that cannot meet the phosphate demand necessary for skeletal mineralization (Figure 96-2).
Intake
Mature human milk contains approximately 260 mg of calcium per liter.42 Considering the possibility of a lower efficiency (fraction) of calcium absorption from infant formulas compared with human milk, the guidelines of the Food and Drug Administration, the Life Sciences Research Office (LSRO),47 and those of the Scientific Committee on Food of the European Commission for full-term infants during the first 6 months of life recommended a content of 50 to 140 mg of calcium per 100 kcal.6 It should be noted that there are no comparisons of calcium absorption performed in the United States for infant formulas and human milk at the same calcium content, as the calcium content of human milk is uniformly less than the minimum mandated for infant formulas by the Infant Formula Act.42 Typical 24-hour intake and retention values for human milk-fed infants is shown in Figure 96-3.
The requirements for enteral calcium intake in preterm infants were reconsidered in a recent American Academy of Pediatrics (AAP) statement.3 The AAP recommended a range of calcium intake of 150 to 220 mg/kg per day consistent with the LSRO recommendations for infants receiving 120 kcal/kg per day of feeding.47 The AAP recommendations are slightly above those of the European authorities (Table 96-2).6 In the United States, fortified human milk or preterm formula generally provides about 180 to 230 mg/kg per day of calcium at usual intakes (Table 96-3). There is no evidence that small variations among formulas within a class (e.g., different brands of preterm formulas marketed in the United States) in amount or source of calcium have a biologically meaningful impact on preterm infants.
TABLE 96-2
Recommendations for Enteral Nutrition for VLBW Infants
Calcium (mg/kg per day) | Phosphorus (mg/kg per day) | Vitamin D (IU/day) | |
Tsang et al. (2005) | 100-220 | 60-140 | 150-400 |
Klein (2002) | 150-220 | 100-130 | 135-338† |
Agostoni (2010) | 120-140 | 65-90 | 800-1000 |
Abrams and AAP CON (2013) | 150-220 | 75-140 | 200-400 |
†90-125 IU/kg (total amount shown is for 1.5-kg infant).
From Abrams SA and the Committee on Nutrition, AAP. Calcium and vitamin D requirements of enterally fed preterm infants. Pediatrics. 2013;131:e1676-1683.
TABLE 96-3
Intakes of Calcium, Phosphorus, and Vitamin D from Various Enteral Nutrition Feedings at 160 mL/kg per day Used in the United States
Unfortified Human Milk* (20 kcal/oz) | Fortified Human Milk* (24 kcal/oz) | Preterm Formula(24 kcal/oz) | Transitional Formula(22 kcal/oz) | |
Calcium (mg/kg) | 37 | 184-218 | 210-234 | 125-144 |
Phosphorus (mg/kg) | 21 | 102-125 | 107-130 | 74-80 |
Vitamin D (IU/day)† | 2.4 | 283-379 | 290-468 | 125-127 |
*Human milk data based on mature human milk.
†Based on an infant weighing 1500 g.
Data from American Academy of Pediatrics, Committee on Nutrition, Kleinman RE, ed. Pediatric nutrition handbook. 6th ed. Elk Grove Village, IL: American Academy of Pediatrics; 2009, and Abrams SA and the Committee on Nutrition, AAP. Calcium and vitamin D requirements of enterally fed preterm infants. Pediatrics. 2013;131:e1676-1683.
Phosphorus Physiology
Unlike calcium, phosphorus is present in the soft tissues, mainly in the form of phosphate esters, and in extracellular fluid in the form of inorganic phosphate ions. This represents about 15% of the whole-body content. Given its widespread distribution, phosphorus plays a critical role in many biologic processes, including energy metabolism, membrane composition, nucleotide structure, cellular signaling, and bone mineralization. It is therefore not surprising that a deficiency of phosphorus results in clinical disease, including muscle weakness, impaired leukocyte function, and abnormal bone metabolism.
Serum Phosphorus
In serum, about two thirds of phosphorus is organic (lipid phosphorus and phosphoric ester phosphorus) and one third inorganic. In routine clinical practice, only inorganic phosphorus is measured. About 85% of the inorganic phosphorus is ionized, circulating as monohydrogen or dihydrogen phosphate; 5% is complexed with sodium, magnesium, or calcium; and 10% is protein bound. The serum concentration is conventionally expressed as the mass of the elemental phosphorus (mmol/L or mg/dL). In contrast to calcium, serum phosphorus concentration varies widely depending mainly on intake and renal excretion, but is also influenced by age, gender, pH, and a variety of hormones. At birth the mean serum phosphorus concentration is relatively low (2.6 mmol/L or 6.2 mg/dL) but thereafter rises rapidly to reach a typical value of 3.4 mmol/L, or 8.1 mg/dL, owing to both endogenous phosphorus release and low renal excretion.54,84 The diet partially determines the serum phosphorus content, with higher values usually seen in infant formula compared to human milk–fed infants. Serum phosphorus is inversely related to serum calcium concentration and to age during childhood. Hypophosphatemia prevents apoptosis in the hypertrophic cells in the growth plate. In the absence of apoptosis, the hypertrophic cells accumulate in the growth plate and form the rachitic bone. It follows that the primary common denominator of rickets is hypophosphatemia, thus the diagnosis of rickets should be based substantially on the etiologies of hypophosphatemia, although in preterm infants with rickets it is clear that significant deficiencies of both calcium and phosphorus occur.90
Placental Transport
During pregnancy, there is transfer of phosphorus from the mother to the fetus that reaches a peak rate of 60 to 75 mg/kg per day during the third trimester; 75% is retained for bone mineralization, and 25% is retained in other tissues. The transplacental transport of phosphorus is an active process against a concentration gradient and is sodium dependent. Both 1,25(OH)2D3 and fetal PTH may be involved in the regulation of placental phosphorus transfer.95
Intestinal Absorption
Intestinal phosphorus absorption takes place primarily in the duodenum and jejunum. It occurs by two mechanisms: an active, sodium-dependent transcellular process localized to the mucosal surface by sodium phosphorous cotransporter 2B (NaPi 2B), and passive diffusion through the paracellular pathway. It depends on both the absolute amount of dietary phosphorus and the relative concentrations of calcium and phosphorus (an excessive amount of either can decrease the absorption of the other). 1,25(OH)2D3 stimulates NaPi 2B expression and active phosphorus absorption. In otherwise healthy intestines, the efficiency of this absorption is high (close to 90% of intake) regardless of the type of feedings provided to the infant.
Renal Excretion
The three major factors that control phosphorous reabsorption are high PTH activity, high FGF (fibroblast growth factor)23 activity, and renal defects that lead to inorganic phosphate (Pi) wasting. Hallmarks of high PTH activity are hypophosphatemia, phosphaturia, disturbance in vitamin D metabolism, and low calcium. The hallmarks of high FGF23 activity are hypophosphatemia and phosphaturia with inappropriately low 1,25-OH2D, and the hallmarks of renal rickets are hypophosphatemia and phosphaturia with high circulating 1,25-OH2D.
The kidney contributes to a positive phosphate balance during growth by the reabsorption of a relatively high fraction of filtered inorganic phosphate (99% in neonates, 95% in infants fed human milk, and 80% in adults). Preterm infants have an increased fractional excretion of phosphate and are at a greater risk for developing signs and symptoms of phosphate deficiency. The bulk of filtered phosphate is reabsorbed in the proximal tubule through sodium-dependent transporters, the Na-phosphate cotransporters 2A and 2C. A change from human milk with a low phosphate content to a formula with a higher content is associated with a rapid down-regulation of Na-phosphate cotransporters mRNA and protein in the brush border membrane.
Because intestinal phosphorus absorption is very efficient and fairly unregulated, renal phosphorus excretion plays an important role in maintaining its balance. Filtered load depends on the plasma phosphorus level and glomerular filtration rate (GFR). Tubular reabsorption is an active and saturable process that gives rise to a maximal rate of tubular reabsorption (Tm). There is a plasma minimal threshold below which phosphorus reabsorption is almost complete and urinary excretion close to zero, and a maximal threshold above which all tubular reabsorptive systems are saturated, so each additional increment in filtered load is associated with a parallel increment in excretion.54 In preterm infants, the minimal and maximal threshold levels are 1.75 mmol/L (5.4 mg/dL) and 2.45 mmol/L (7.6 mg/dL), respectively (Figure 96-4). The three main factors responsible for Pi readsorption by the sodium/Pi cotransporter in the proximal tubule are urinary phosphorus, PTH, and FGF23. Renal handling of Pi is regulated by hormonal and nonhormonal factors. Changes in urinary excretion of Pi are almost invariably mirrored by changes in the apical expression of NaPi-IIa and NaPi-IIc in proximal tubules. Phosphate deprivation increases NaPi-IIa and NaPi-IIc expression in the proximal tubule. Both NaPi-IIa and NaPi-IIc, the main sodium/Pi cotransporters, are regulated in a similar fashion by PTH, FGF23, and dietary phosphate. Both PTH and FGF23 accelerate the cotransporter endocytosis and FGF23 also accelerates cotransporters’ degradation in the lysosome.63

During early postnatal life, the phosphate response to PTH is blunted, whereas PTH increases tubular calcium reabsorption. Together, these actions result in the retention of both calcium and phosphate in infants, which is favorable for growth and bone mineralization.
The level of FGF23 in cord blood is low, which might be caused by the low expression of FGF23 in fetal tissues. Measuring soluble α-Klotho and FGF23 levels in the cord blood reveals a negative correlation between the two.66 The low FGF23 levels promote a relative hyperphosphatemia and the relative increase in 1,25(OH)2D3 promotes bone mineralization during early rapid growth in the neonatal period.
FGF23 functions principally as a phosphaturic factor. It is secreted by osteocytes and osteoblasts in response to high serum phosphate levels and 1,25(OH)2D3. In the osteoblast, FGF23 post-translation undergoes O-glycosylation by GALNT 3. The glycosylation protects the molecule from degradation. Nonglycosylated FGF23 may be targeted for degradation by subtilisin/furin-like endopeptidases. Impaired FGF23 synthesis or action, owing to FGF23 gene mutations; mutations in GALNT 3; or mutations in Klotho, which is required for the conversion of FGFR1(IIIc) into the FGF23 receptor, leads to severe hyperphosphatemia and tumoral calcinosis.91
Absorbed phosphate enters the extracellular phosphate pool, which is in equilibrium with bone and soft tissue. In growing infants, the amount of phosphorus excreted is less than the net amount absorbed owing to the deposition of phosphorus in soft tissues and bone. In infants, phosphorus will preferentially go to soft tissue with a weight-to-weight nitrogen-to-phosphorus ratio of 15 : 1 and to bone with a weight-to-weight calcium-to-phosphorus ratio of 2.15 : 1. The residual phosphorus constitutes the renal phosphorus load influencing plasma concentration and urinary excretion. In the face of a limited total phosphorus supply, bone mineral accretion may be limited, leading to significant calcium excretion associated with very low urinary excretion of phosphorus. This particular situation is illustrated in Figure 96-2, showing calcium and phosphorus metabolism in term infants fed human milk or formula.
By dividing clearance / clearance, the time and volume are redundant, and
(Up, Urinary phosphorus; Scr, serum creatinine; Sp, serum phosphorus; Ucr, urine creatinine; Pi, inorganic phosphate)
The normal range for TRP is 80% to 90%; low values indicate phosphaturia, such as in hyperparathyroidism or hypophosphatemic rickets; high values designate enhanced Pi reabsorption, such as in hypo- or pseudohypoparathyroidism. In the presence of abnormally high or low serum Pi, renal reabsorption decreases or increases, respectively. The TRP becomes therefore a lesser value in such conditions, and TmPO4/GFR is calculated. TmPO4, the tubular threshold for Pi reabsorption, reflects the highest serum Pi levels that will result in complete reabsorption of the filtrated Pi load. The nomogram developed for that purpose almost four decades ago is still a useful bedside tool (Figure 96-5).94

Intake
Mature human milk contains about 140 mg of phosphorus per liter. To avoid the occurrence of hypocalcemia or hypercalcemia resulting from an unbalanced diet containing the minimal calcium combined with the maximal phosphorus content (or the inverse, the Life Sciences Research Office and the Scientific Committee on Food of the European Commission recommend that the calcium-to-phosphorus ratio be maintained at more than 1.1 : 1 but less than 2.0 : 1.6,47 Recently, the AAP recommended intakes of 75 to 140 mg/kg per day of phosphorus for very low birth weight (VLBW) infants.3
Magnesium Physiology
The skeleton represents the largest magnesium store (60%), which is divided into two compartments: one firmly bound to apatite and nonmobilizing, and the other absorbed to the surface of the mineral crystals and contributing to magnesium homeostasis. The remaining magnesium is distributed in skeletal muscle, the nervous system, and other organs with a high metabolic rate. Magnesium is the second most abundant intracellular cation, playing a crucial role in many physiologic functions. It is critical in energy-requiring metabolic processes, protein synthesis, membrane integrity, nervous tissue conduction, neuromuscular excitability, muscle contraction, hormone secretion, and intermediate metabolism.
Serum Magnesium
In serum, about one third of the magnesium is bound to protein, mainly albumin; the remaining two thirds is ultrafilterable, being about 92% free and 8% complexed to citrate, phosphate, and other compounds.53 The plasma magnesium concentration is elevated in preterm and term infants (0.8 ± 0.16 mmol/L, or 2.0 ± 0.4 mg/dL) and decreases soon after infancy to adult values.49 The sum of ionized and complexed magnesium constitutes its diffusible or ultrafilterable form. Although the concentration of this form of magnesium in plasma remains almost constant (0.45 ± 0.1 mmol/L, or 1.1 ± 0.2 mg/dL), the proportion of this diffusible form in relation to total magnesium increases progressively with age (preterm newborn infants, 52%; term newborn infants, 62%; infants, 66%).
Placental Transport
Magnesium freely crosses the placental barrier and accumulates in the fetus mainly during the first trimester of pregnancy. Placental transfer continues throughout gestation, at a daily rate of 3 to 5 mg. The transfer of magnesium across the placenta depends on an active transport mechanism different from that of calcium, which is necessary to maintain higher fetal than maternal concentrations. Situations of magnesium excess or deficiency in the mother are also reflected in the fetus.61
Intestinal Absorption
About 40% of ingested magnesium is absorbed in the intestine, mainly in the proximal parts. There are two mechanisms of absorption: one is passive and the other active and saturable. Passive absorption occurs by means of a paracellular pathway, following a favorable electrochemical gradient as a function of water and solute movement, and appears to be proportional to dietary intake. Regulated, active transport depends on a saturable carrier present in the luminal membrane and operates only under conditions of low magnesium intake. The factors regulating the intestinal absorption of magnesium are largely unknown. The magnesium concentration in the digestive tract is the most important determinant of the amount of magnesium absorbed, nevertheless. Substances that increase magnesium solubility favor its absorption, whereas substances that form insoluble complexes decrease its likelihood. Magnesium absorption in the gut is not regulated by 1,25(OH)2D3.
Renal Excretion
The kidney plays a major role in magnesium homeostasis. It conserves magnesium in response to a deficiency and increases excretion in proportion to the load presented to the kidney. About 70% to 80% of serum magnesium is filtered through the glomerular membrane, but only 5% to 15% of the filtered magnesium is reabsorbed along the proximal tubule, which is considerably less than the amount of fractional reabsorption of sodium or calcium. The primary site for magnesium reabsorption is the thick ascending limb of the loop of Henle, where about 65% of the filtered magnesium is reabsorbed. When the magnesium intake is severely restricted in humans with normal kidney function, urine output decreases. Supplementing a normal intake increases urinary excretion without altering normal serum levels as long as renal function is normal and the amounts given are not excessive. Overall, the tubular reabsorption of magnesium is a process that is quantitatively limited; the maximal rate of this reabsorption appears to be lower than that of calcium and phosphorus.57
Intake
Mature human milk contains about 26 mg of magnesium per liter.43 Guidelines for infant formulas during the first 6 months of life recommend minimal and maximal contents of 4 to 5 mg and 15 to 17 mg/100 kcal, respectively. For preterm infants, the recommendations are based on fetal accretion rates, and values similar to those at term have been suggested (6.8 to 17 mg/100 kcal and 7.9 to 15 mg/kg per day, respectively).6,43,47,72,92
Hormonal Regulation of Mineral Metabolism
Parathyroid Hormone
The parathyroid glands, through the secretion of PTH, regulate serum calcium concentrations and bone metabolism. PTH is synthesized as a larger (115-amino acid) precursor (prepro-PTH), but is stored and secreted mainly as an 84-amino acid peptide, with the 1-34,N-terminal portion conferring bioactivity.56
Effects
Parathyroid hormone increases serum calcium concentrations directly by increasing bone resorption and renal calcium reabsorption and indirectly by increasing renal synthesis of 1,25(OH)2D3, thereby increasing intestinal calcium absorption. In addition, PTH lowers serum phosphorus concentrations through its phosphaturic action on the renal proximal tubule. This action minimizes the possibly adverse effect of hyperphosphatemia related to bone resorption on calcium homeostasis.
Regulation of Hormonal Secretion
Serum calcium concentration regulates PTH secretion; high concentrations inhibit the secretion of PTH, and low concentrations stimulate it. Low or falling serum calcium concentrations act within seconds to stimulate PTH secretion, initiated by means of a calcium-sensing receptor on the surfaces of parathyroid cells. This receptor is expressed in the parathyroid glands where it regulates PTH secretion and in the kidneys where it regulates tubular calcium reabsorption. Decreases in ionized calcium of as little as 0.4 mmol/L stimulate PTH secretion, and increases suppress it. Acute decreases in magnesium concentrations also stimulate PTH secretion, and increases depress it. However, chronic magnesium deficiency paradoxically decreases PTH secretion, probably by altering the calcium-sensitive, magnesium-dependent adenylate cyclase involved in PTH secretion.
Vitamin D and its metabolites 25-OHD and 1,25-OH2D, acting through vitamin D receptors, decrease the level of PTH mRNA. The human calcium receptor gene contains six exons and is located at 3q13.3-21 (Online Mendelian Inheritance in Man, OMIM, 601199). In the parathyroid gland, the calcium receptor mediates inhibition by extracellular Ca2+ concentration of PTH secretion, of PTH gene expression, and of parathyroid cellular proliferation. In the kidney, the receptor mediates the direct inhibition of the reabsorption of divalent cations in the thick cortical ascending limb of the loop of Henle.78 Inactivating or activating mutations of this receptor cause inherited human conditions.
Both activating and inactivating calcium-sensing receptor (CaSR) mutations have been identified. Loss-of-function CaSR mutations lead to familial hypocalciuric hypercalcemia, which is an autosomal dominant condition usually characterized by mild degrees of hypercalcemia, inappropriately normal PTH levels, and low (or inappropriately low) urinary calcium excretion.70 Gain-of-function CaSR mutations are associated with dominant hypocalcemic hypercalciuria. Some cases of nonfamilial idiopathic hypoparathyroidism may also be caused by gain-of-function CaSR mutations.
Fetal Parathyroid Function
Maternal immunoreactive PTH, like other polypeptide hormones, does not cross the placenta. Concentrations of PTH increase from the first to the second trimester, declining again in the third trimester to the levels of the first trimester. However, PTH is significantly increased postpartum.12 In contrast, PTHrP concentrations increase continuously throughout pregnancy and lactation. Human parathyroid glands are functionally active as early as 12 weeks of gestation. However, fetal parathyroid glands are functionally suppressed by high intrauterine calcium concentrations, and PTH levels in cord blood are frequently below the detection limit.80 Loss of PTH has no effect on overall fetal growth (crown-rump length, weight); loss of PTHrP has a modest effect on fetal growth; loss of PTH and PTHrP has a more pronounced effect on fetal growth; loss of the PTH1 receptor causes the most marked fetal growth restriction.
Neonatal Parathyroid Function
After birth, with the abrupt termination of the maternal calcium supply, serum calcium in the newborn decreases, and serum PTH increases correspondingly. Both term and preterm infants may show a PTH response to falling serum calcium. Infants with VLBW have a decreased PTH surge compared with full-term infants. Infants of diabetic mothers may have impaired PTH production during the beginning days of life. Infants with severe perinatal depression may also have decreased PTH responses to hypocalcemia.
Parathyroid Hormone Reference Values
Current assays for serum PTH are conducted at two sites that are designed to detect both amino-terminal and carboxy-terminal epitopes of the peptide. PTH molecules that are reactive in these two-site immunoassays are considered intact.
In full-term newborn infants, serum PTH concentrations tend to be low in cord blood, but increase within the first 48 hours of life in response to the decrease in serum calcium. In preterm infants, PTH concentrations increase immediately after birth, indicating that, in them, the secretion of the hormone responds physiologically to the hypocalcemic stimulus. This increase in PTH concentration could be blunted when premature infants receive calcium by infusion, with the calcium load buffering the postnatal depression of serum calcium. By day of life 10, the serum concentrations of intact PTH return to euparathyroid values (Table 96-4). The multiplication factor for serum PTH from picograms per deciliter to picomoles per liter (pg/dL to pmol/L) is 0.11.
TABLE 96-4
Evolution of Intact Parathyroid Hormone (1-84), Carboxy-Terminal Parathyroid Hormone, and Vitamin D–Binding Protein Concentrations in 15 Preterm Infants
PTH(1-84) (pmol/L) | cPTH (pmol/L) | DBP (µmol/L) | |
Cord serum | 11 ± 3 | 48 ± 8 | 4.43 ± 0.37 |
Day 1 | 66 ± 11* | 125 ± 15* | 4.40 ± 0.34 |
Day 2 | 87 ± 11* | 168 ± 5* | 4.96 ± 0.23 |
Day 5 | 67 ± 9* | 152 ± 16* | 6.21 ± 0.26* |
Day 10 | 23 ± 4 | 69 ± 6 | 6.03 ± 0.30* |
Day 30 | 38 ± 7 | 80 ± 11 | 5.16 ± 0.23 |
cPTH, Carboxy-terminal PTH; DBP, vitamin D-binding protein; PTH, parathyroid hormone.
*Significantly different from cord serum, P < .05.
Data from Salle BL, et al. Perinatal metabolism of vitamin D. Am J Clin Nutr. 2000;71:1317S.
Vitamin D
Synthesis and Metabolism
Vitamin D is synthesized endogenously in the skin (cholecalciferol or vitamin D3) after sunshine exposure to high-energy ultraviolet photons (ultraviolet B, 290 to 315 nm) or is absorbed from dietary sources in the duodenum and jejunum as either vitamin D3 (from animal sources) or vitamin D2 (ergocalciferol, from vegetable sources). Regardless of its origin, vitamin D2 and D3 are transported bound to the vitamin D–binding protein to the liver, where it is hydroxylated at carbon 25 to form vitamin D3 (25(OH)D3, or calcidiol), the most abundant vitamin D metabolite. The generation of calcidiol is regulated by CYP2R1.22 Circulating concentrations of 25(OH)D3 provide a useful index of vitamin D status (reflecting both dietary intake and sunshine exposure). Subsequently, 25(OH)D3 is further hydroxylated at carbon 1 to form the final active metabolite, 25-hydroxyvitamin D3-1α-hydroxylase (1α-hydroxylase), which is classically expressed in the kidney. However, 1,25(OH)2D3 can also be synthesized by various cells, including monocytes and skin cells, as well as by the placenta during pregnancy.97
Effects
Normal vitamin D status is necessary to maintain calcium and phosphorus homeostasis. The effects of 1,25(OH)2D3 (calcitriol) on target tissues are initiated by its binding to a steroid receptor (vitamin D receptor) distributed in numerous tissues, leading to the synthesis of a variety of proteins. Therefore 1,25(OH)2D3 acts on the small intestine, increasing the absorption of calcium and phosphorus by the synthesis of calcium-binding (calbindin-D) proteins; on bone, mobilizing calcium and phosphorus by increasing the number of osteoclasts; and on the kidney, increasing calcium reabsorption in the distal nephron segments by increasing the expression of the epithelial calcium influx channel.
Regulation of Secretion
Renal 1-hydroxylation, which leads to the active metabolite, is tightly regulated. The main factors increasing the synthesis of calcitriol through stimulation of renal 25(OH)D3-1α-hydroxylase are PTH, PTHrP, hypocalcemia, hypophosphatemia, and other hormonal factors, such as IGF-1, estrogen, prolactin, and growth hormone. The production of calcitriol is inhibited by elevated serum levels of calcium and phosphorous and by FGF23.71
Fetal Vitamin D Function
Serum 25(OH)D concentration depends on vitamin D intake and production. The production of vitamin D is influenced by geographic location, season, skin pigmentation, and latitude. Vitamin D deficiency is very common during pregnancy, especially in areas with a prolonged winter season.45 It is lower in African-American pregnant women in contrast to Caucasian pregnant women. In the United States, nearly half of African-American pregnant women are vitamin D deficient with a serum 25(OH)D less than 37.5 nmol/L, as opposed to less than 30% of Caucasian women.17,42
Cord concentrations of the major vitamin D metabolites are consistently lower than those measured in the mother’s serum. Placental venous 25(OH)D concentrations correlate significantly with those found in the maternal circulation, suggesting that calcidiol easily diffuses across the placental barrier and that the vitamin D pool of the fetus depends entirely on that of the mother. Most of the 1,25(OH)2D in fetal plasma is owing to fetal kidney activity, as suggested by studies in fetal plasma from infants with renal agenesis.
In undernourished populations with vitamin D deficiency, osteomalacia in the mother and abnormal skeletal metabolism in the fetus and infant have been reported. Infants of severely malnourished mothers may be born with rickets and can suffer fractures during the neonatal period. Therefore, bone mass of the newborn infant may be related to the vitamin D status of the mother. Comparison of the results of dual-energy x-ray absorptiometry (DEXA) from different countries shows that infant whole-body bone mineral content values are lower in countries in which milk products are not supplemented with vitamin D than in those in which milk products are supplemented. In contrast, vitamin D supplementation of malnourished mothers results in improved growth of the fetus and child in terms of both birth weight and subsequent linear growth during infancy. Moreover, maternal vitamin D status during pregnancy may have influence on the fetal skeletal development starting as early as 19 weeks’ gestation and can persist long after infancy.55 At the age of 9 years, children whose mothers had deficient or insufficient concentrations of 25-OHD in late pregnancy had a reduced bone size and bone mineral content.44
Maternal vitamin D deficiency and insufficiency during pregnancy have been associated with an increased risk for preeclampsia, insulin resistance and gestational diabetes mellitus, and primary cesarean delivery.4,23,59
Current guidelines from the Institute of Medicine as endorsed by the American Academy of Pediatrics recommend a total dietary and supplement intake (RDA) of 600 IU/day of vitamin D during pregnancy and lactation.11,42 Although some, including the Canadian Paediatric Society,35 have suggested higher doses may be preferred, more safety and efficacy research is needed regarding high-dose vitamin D supplementation in pregnancy and lactation.
Neonatal Vitamin D Function and Recommendations
Serum or plasma 25(OH)D concentration is a useful vitamin D biomarker reflecting vitamin D supply (exposure) over a period of time. Nevertheless, several surveys show a high rate of poor maternal vitamin D status throughout the world, particularly in countries without vitamin D supplementation, with poor sun exposure, extensive clothing, or with deeply pigmented skin. Thus, the rate of cord blood vitamin D deficiency (<20 nmol/L; <8.3 ng/mL) may reach up to 70% in European populations.68
Vitamin D content in human milk is usually low.38,42 A daily intake of up to 400 IU (10 micrograms/day) of vitamin D is recommended by the Institute of Medicine and endorsed by the American Academy of Pediatrics for all infants beginning soon after birth.11 In addition, vitamin D is metabolized in the liver, and anticonvulsants such as phenobarbital and diphenylhydantoin increase its hepatic catabolism and requirements. There are some questions about what is meant in terms of timing of initiation by the available statements. In most practices, it is considered ideal to begin healthy full-term infants who are being partly or wholly breastfed, on vitamin D supplements prior to their hospital discharge or not later than a week of age for those with longer initial hospitalizations.
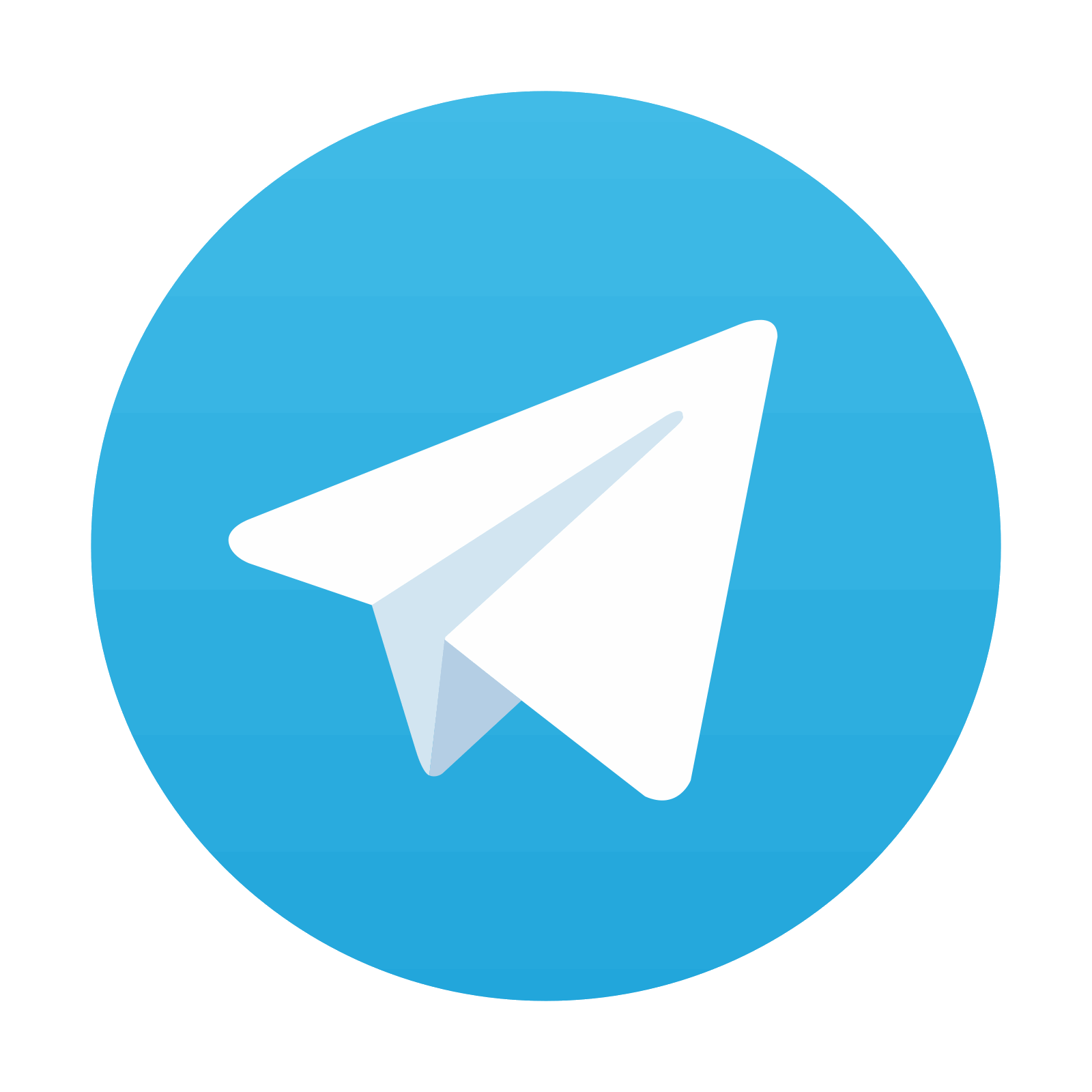
Stay updated, free articles. Join our Telegram channel
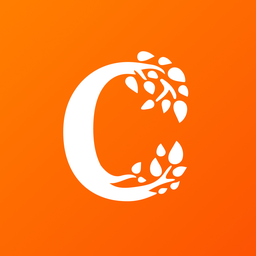
Full access? Get Clinical Tree
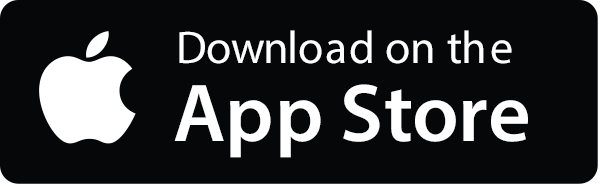
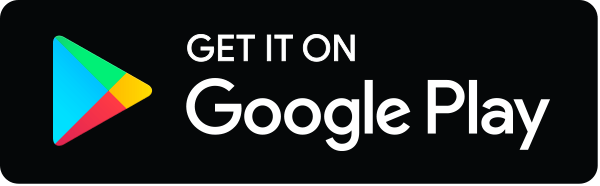