Developmental Immunology
John T. Benjamin, Olachi J. Mezu-Ndubuisi and Akhil Maheshwari
Birth represents a functional watershed in the developing immune system. In utero, the fetus is exposed to a constant barrage of “foreign” antigens that are derived mainly from the mother, and must downregulate its immune responses to survive. However, after birth, the neonatal immune system is exposed to a new, more varied set of antigens and must evolve dichotomous responses to simultaneously “contain” microbial populations on various cutaneous and mucosal surfaces, and also develop tolerance to commensal microbes and dietary macromolecules. Although some components of the neonatal immune system perform adequately and at par with adults, immaturity of several major arms of immunity results in a developmentally regulated, transient state of immunodeficiency during early infancy. This chapter highlights major strengths and limitations of the neonatal immune system.
The immunologic system is broadly comprised of two major host defense mechanisms: innate, or nonspecific, immune mechanisms and acquired, or specific, immune mechanisms. By definition, innate immunity includes host defense mechanisms that operate effectively without prior exposure to a microorganism or its antigens. Some of these mechanisms include physical barriers, such as intact skin and mucous membranes, and chemical barriers, such as gastric acid and digestive enzymes. Beneath these important protective layers lie phagocytic cells, which constitute the first line of host defense against any microbes that breach the cutaneous and mucosal barriers. Soluble plasma and tissue proteins serve to amplify the function of the phagocytic cells as innate immune effectors. Acquired, or specific, immunity comprises primarily the cell-mediated (T lymphocyte) and humoral (B lymphocyte and immunoglobulin) systems.
Both innate and acquired immune mechanisms are necessary for an individual to be fully immunocompetent. These systems are intimately interrelated and interdependent. Phagocytes such as neutrophils and the cells of the monocytes-macrophage lineage are important effectors of innate immunity because these cells function to ingest and clear microbial pathogens from normal tissues. Monocytes and macrophages also process microbial antigenic material for presentation to T lymphocytes, a pivotal step in the initiation of a specific immune response.
Overview of Hematopoiesis
See Chapter 88. During fetal development, hematopoiesis first begins in the extra-embryonic mesoderm of the yolk sac (YS) and later shifts to the liver and then to the marrow.1 Yolk sac hematopoiesis continues from the 3rd through the 8th to 10th week. Hepatic hematopoiesis begins in the 5th week, continues through 20 to 24 weeks, and is mainly erythropoietic; although myeloid progenitors are abundant in the liver, mature neutrophils are not identifiable.2 Hematopoiesis is observed in the marrow by the 11th week, when committed granulocytic progenitors are first seen in the clavicular marrow.3 Mature neutrophils are seen in the marrow in the 14th week.4 As gestation proceeds to the 20th week, the bone marrow becomes the major site of hematopoiesis and thereafter remains the primary reservoir for replenishing circulating populations of immune cells.5
All the cellular components of the immunologic system have limited life spans, and the cells must be constantly replenished from a pool of undifferentiated precursor cells derived from a pluripotent stem cell.6 Through mechanisms that are not well understood, pluripotent stem cells are stimulated to divide and differentiate into committed progenitor cells that mature into circulating blood cells. Progenitor expansion and differentiation are normally driven and regulated by a variety of growth factors supplied by fibroblasts, endothelial cells, and macrophages present in the microenvironment of the hematopoietic stem cells.7,8 Emerging evidence also indicates a role for the local extracellular matrix; specific matrix-associated glycoproteins may favor one cellular lineage over another. For instance, the glycoprotein hemonectin is involved in granulocytic development, whereas fibronectin is associated with erythroid differentiation.9
Pluznick and Sachs10 and Bradley and Metcalf11 first cultured hematopoietic progenitor cells in semisolid media and named these progenitors colony-forming units (CFUs). Colony-forming units producing a mixture of granulocytes, macrophages, and erythrocytes were called CFU-MIX, whereas the CFUs producing granulocytes, macrophages, erythrocytes, and megakaryocytes were called CFU-GEMM. Other CFUs gave rise to only one to two cell lineages: CFU-G produced only neutrophils, CFU-M produced monocytes, CFU-GM gave rise to both neutrophils and monocytes, and CFU-Meg produced only megakaryocytes.12 More recently, development of monoclonal antibodies that recognize cell surface molecules expressed on hematopoietic stem cells has permitted the isolation and characterization of these cells.13
Innate Immunity
Cellular Components
The most primitive host defense mechanism involves the ingestion and killing of bacteria and other microorganisms by phagocytic cells. Polymorphonuclear neutrophils (PMNs), monocytes, and macrophages are the major cell types that accomplish this aspect of host defense. Natural killer (NK) cells are also important components of the innate immune system, but these cells kill invading pathogens by nonphagocytic mechanisms. All these cell types can eliminate pathogens from the host, but do so more efficiently when the pathogens are opsonized, or coated, by complement components and other soluble proteins of the innate immune system. Similarly, nonphagocytic methods such as lysis of infected cells by NK cells, PMNs, and monocytes are also augmented in the presence of specific antibody to the target organism. This section provides an overview of the phagocyte and NK cell systems.
Polymorphonuclear Neutrophil System
Kinetics of Production and Circulation.
In the human fetus, granulocytopoiesis takes place almost exclusively in the bone marrow.14 The PMN system arises from two major hematopoietic progenitors, the CFU-mix and the CFU-GEMM.12 In the bone marrow, the neutrophil cell lineage includes early precursors, which have a capacity for four to five cell divisions (the neutrophil proliferating pool or the NPP), and the later, post-mitotic stages that are in process of differentiation (neutrophil storage pool or NSP).15 In adults, the NPP contains about 2 × 109 cells/kg body weight and the NSP contains about 6 × 109 cells/kg body weight.16 The NPP and NSP together contain nearly 90% of all neutrophils in the body. The NSP constitutes a reserve pool of cells that may be rapidly mobilized into the circulation in response to inflammation.4
Positive and negative regulators of PMN production have been identified. Positive regulatory factors are interleukin (IL)-3, granulocyte colony–stimulating factor (G-CSF), and the granulocyte–macrophage colony–stimulating factor (GM-CSF). Several negative regulators of PMN production have also been identified, including the interferons (IFNs), transforming growth factor-α, macrophage inflammatory protein-1α (CC motif-ligand 3), prostaglandins, and lactoferrin and other iron-binding proteins. Although the mechanisms by which PMNs are released from the marrow under physiologic conditions are unclear, increasing evidence indicates an important role for the chemokine receptor CXCR4.17 During inflammation, IL-1, tumor necrosis factor (TNF), epinephrine, and complement fragments are known to stimulate PMN release from the bone marrow.17 Once released from the marrow, mature PMNs circulate for approximately 6 to 8 hours before migrating into tissues, where these cells survive for about an additional 24 hours. Although in the bloodstream, half of the PMNs are attached to the microvascular endothelium, mainly in the lungs, and the other half are free in circulation. After PMNs emigrate from the blood vessels and enter a tissue, they do not re-enter blood vessels, but age and die in the tissue. The actual site of PMN clearance is unknown, but macrophages ingest and degrade senescent apoptotic PMNs in vitro.4
Mature PMNs are first identified in the fetal bone marrow at approximately 14 weeks of gestation.5 By 22 to 23 weeks of gestation the circulating PMN count has increased but remains lower than in term newborns. The fetal blood contains a 10- to 50-fold higher concentration of CFU-GM than adult blood,18 but the fetus has a much smaller total pool of neutrophil progenitors.19 However, the actual number of CFU-GMs in the human neonate is unknown. In the rat, the total-body CFU-GM pool was approximately 10% by weight of that of adult rats. One explanation for the high concentration of circulating progenitors is that these stem cells may have left one hematopoietic environment (the liver) for another (the bone marrow). Consistent with data on low neutrophil mass in the fetus, studies in premature infants show low plasma concentrations of soluble CD16, which is released from apoptotic neutrophils and has been used as a surrogate measure for the total neutrophil mass in the body.20
In the midgestation fetus and preterm infant, the NSP is very small in size and can be readily exhausted during sepsis.16 The NPP is also smaller, about one-tenth the size (per kg body weight) of adults.21 Interventions such as administration of recombinant G-CSF, GM-CSF, and corticosteroids can release neutrophils from the NSP.22–24 Epinephrine can rapidly release marginated neutrophils into circulation for about 30 to 45 minutes.25 A mild demargination response has also been reported in preterm infants after red blood cell transfusions.26
Normal Polymorphonuclear Neutrophil Concentrations and Neutropenia.
Circulating concentrations of PMNs increase dramatically at birth, peak at 12 to 24 hours, and then decline slowly by 72 hours to remain stable during the rest of the neonatal period. Blood PMN concentrations can be interpreted using one of several available reference ranges.27–29 The absolute neutrophil count (ANC) can be calculated from a routine complete blood count (CBC) by multiplying the white cell count with the sum of segmented and band neutrophil percentages on the differential count. Manroe and colleagues30 were the first to compile reference ranges for blood neutrophil concentrations in neonates using data from a cohort of 434 neonates born at 38.9 ± 2.4 weeks’ gestation. They showed that the neutrophil counts peaked at 12 to 24 hours with 95% confidence limits of 7800 to 14,500/µL and then stabilized at a lower value of 1750 by 72 hours of life. A stable upper limit was achieved at 6.6 days of age. Although these ranges were useful for term and late-preterm neonates, these did not include many preterm infants.31–34 To address this deficiency, Mouzinho and colleagues29 compiled ANC values from 1788 CBCs drawn from 63 neonates born at 29.9 ± 2.3 weeks’ gestation. Their revised charts were comparable to those from Manroe and colleagues near the upper limits of blood neutrophil concentrations, but showed greater variation at the lower limit. In a more recent study, Schmutz and associates27 compiled a new set of reference ranges for ANCs using data from 30,354 CBCs from neonates born at 23 to 42 weeks’ gestation. Besides the large sample size, a major strength of this study was the use of automated blood counting instrumentation, which allows greater consistency in identification of neutrophils and includes a larger number of cells for each test.35 In the interval between 72 and 240 hours after birth, the ANC ranged between 2700 and 13,000/µL (5th to 95th percentile) for infants greater than 36 weeks’ gestation, between 1000 and 12,500/µL at 28 to 36 weeks, and between 1300 and 15,300/µL at less than 28 weeks’ gestation. The upper limits of ANC in this data set were higher than ranges reported by both Manroe and Mouzinho, which may have been owing to the high altitude at which the participating centers were located.36,37
When searching for the cause of neutropenia (<1500 PMNs/mm3) in infants, a strong suspicion of infection is warranted, although maternal preeclampsia, premature birth, birth depression, intraventricular hemorrhage, and hemolytic disease may result in low peripheral PMN counts. Persistent neutropenia is one feature that is frequently seen in patients who succumb to overwhelming sepsis. Neutropenia in these infants may be caused by increased margination of activated circulating cells or rapid depletion of circulating and bone marrow storage pool PMNs. Failure to provide adequate numbers of PMNs to the sites of microbial invasion may contribute to the risk of overwhelming sepsis in neonates.4
Overview of Polymorphonuclear Neutrophil Function.
The PMN is qualitatively and quantitatively the most effective killing phagocyte of host defense. Numerous coordinated steps are required to attract large numbers of PMNs from the circulating blood into a tissue at the site of microbial invasion (Figure 54-1). During acute inflammation, first mature neutrophils, and then progressively immature cells are released from the bone marrow storage pool into the circulation. The circulating PMNs exit the intravascular compartment to enter tissue sites of inflammation in three steps: margination and rolling on vascular endothelium, attachment to the endothelial cells, and transendothelial migration.38 In the face of microbial translocation across cutaneous or mucosal barriers, a variety of chemoattractants recruit circulating PMNs, including microbial products such as N-formyl-methionyl-leucyl-phenylalanine (f-MLP) and host factors such as CXC chemokines (particularly those expressing the tripeptide sequence glutamic acid-leucine-arginine, such as IL-8), products of the complement system (C5a), lipids such as leukotrienes (LTB4) and hepoxilin-A3, collagen fragments containing the tripeptide sequence proline-glycine-proline, and nuclear matrix proteins (e.g., high-mobility group box-1) that are released during cell death.

Rolling and Adhesion.
Polymorphonuclear neutrophils adhesion to endothelial cells is a crucial step in the recruitment of these leukocytes to inflammatory sites. Although PMNs can adhere to normal or activated vascular endothelium, inflammation is associated with hemodynamic and biochemical changes in blood vessels that facilitate leukocyte adhesion to the vessel endothelial lining. Polymorphonuclear neutrophils flowing through an inflamed venule may transiently adhere to the endothelium to “brake” their flow, which causes the neutrophils to “tumble” or “roll” on the vascular surface. Leukocyte rolling is mediated via the interaction of L-selectin molecules on neutrophils with endothelial glycoproteins called addressins (Figure 54-2): glycosylation-dependent cell adhesion molecule-1 (GlyCAM-1), CD34/sgp90, endomucin, podocalyxin, endoglycan, and the mucosal vascular addressin cell adhesion molecule-1 (MAdCAM-1).39 Endothelial cells also express two selectin molecules, E-selectin/CD62E and P-selectin/CD62P, which interact with the P-selectin glycoprotein ligand-1 (PSGL-1) and E-selectin ligand-1 (ESL-1) on leukocytes.39–42

After a few rolling events, leukocytes slow down and rest on the endothelium. During this phase, the interaction of leukocyte integrins with endothelial receptors results in neutrophil activation and also initiates signaling events in the endothelium, leading to transendothelial migration of leukocytes. Integrins involved in neutrophil trafficking include three heterodimeric proteins, each of which consists of one immunologically distinct “a” subunit (leukocyte functional antigen-1; CD11a, Mac-1; CD11b, p150, 95; CD11c), along with a common “b” subunit (CD18). Monoclonal antibodies directed against CD11b/CD18 inhibit PMN aggregation, spread, chemotaxis, and adhesion to endothelial cells. CD11b/CD18 and CD11c/CD18 serve as complement receptors and mediate complement-coated (fragment iC3b) particle ingestion by PMNs. CD11a/CD18 has been shown to be important in PMN killing of target cells through antibody-dependent mechanisms. Patients with a heritable deficiency of these leukocyte cell surface glycoproteins have recurrent infections characterized by failure to accumulate granulocytes at sites of infection.
Neutrophil adhesion to vascular endothelium and other phagocytes depends on several specific receptor-ligand interactions at the cell surface membrane (see Figure 54-2). Circulating neutrophils usually roll along vascular endothelial cells with transient interactions between neutrophil selectins on the cell surface and counter-receptors on the endothelium. When endothelial cells are activated during inflammation, more selectin counter-receptors are expressed, and additional proteins (intercellular adhesion molecule [ICAM]-1 and [ICAM]-2) of the immunoglobulin supergene family, which serve as ligands for the neutrophil integrin family, are activated. The rolling neutrophil is slowed by the more concentrated interactions of the neutrophil cell surface selectins and the endothelial counter-receptors. Simultaneously increased interactions of neutrophil cell surface integrins with endothelial ICAM-1 and ICAM-2 occur, neutrophil cell surface selectins are shed, and a firm neutrophil-endothelial adhesive interaction is established. After tight adhesion, the PMN begins a process of diapedesis through adjacent endothelial cells and the intact underlying basement membrane.43 Once the PMN has penetrated the basement membrane, the cell migrates from the blood vessel into the area of inflammation. In the tissues, initial random migration (chemokinesis) becomes progressively directed (chemotaxis) toward the nidus of microorganisms along concentration gradients of bacterial products or chemotactic factors.
Adhesion of PMNs to artificial surfaces in vitro is comparable for unstimulated PMNs isolated from neonatal or adult blood. When PMNs from term neonates are stimulated with chemotactic factors, adhesion is greatly diminished, however, compared with cells isolated from adults. More profound deficits are displayed by PMNs from preterm infants. Neonatal PMNs show lower selectin expression, defective shedding of L-selectin,44 and less stimulated mobilization and overall expression of CD11b/ CD18 glycoproteins on the plasma membrane. Other causes of diminished stimulated adherence include impaired capacity to upregulate cell surface chemotactic receptors, lower granular content of lactoferrin, less fibronectin binding to the cell surface, and less shape change (failure to increase significantly overall cell surface area on chemotactic factor stimulation). Downregulation of L-selectin expression on term newborn cord blood granulocytes and monocytes during acute inflammation has been shown, although the pattern and level of shedding vary from neutrophils isolated from adult subjects.
Compared with neutrophils from adults, neutrophils from both term and preterm neonates adhere poorly to endothelium. Neonatal neutrophils have lower selectin and β-integrin expression. In neonates, L-selectin expression is lower at birth than in adults and decreases further during the first 24 to 72 hours.44 Neonatal “stress,” as in perinatal asphyxia, may further reduce L-selectin expression on neutrophils.45 In addition, neonatal neutrophils display defective shedding of L-selectin.44 The combination of lower expression and impaired shedding of L-selectin reduces the frequency of neutrophil rolling events, which are a rate-limiting step in the tissue recruitment of neutrophils. Characteristics of preterm vascular endothelium such as lower P-selectin expression further contribute to these defects in neutrophil recruitment.46
Neonatal neutrophils have lower expression of Mac-1(CD18/CD11b), one of the β2 integrins, which correlates with lower neutrophil-endothelial adherence and transmigration.47 Neutrophils from both preterm and term infants are unable to upregulate Mac-1 expression following stimulation by bacterial products. The absolute Mac-1 content per neutrophil is lower in preterm and term neonates: Mac-1 expression is about 10% of adult levels at 27 weeks, increases to 45% at 36 weeks, is 57% at term, and reaches adult levels in early childhood.48
Transendothelial migration of neutrophils is affected to some degree by deformability of neutrophils. Although initial studies reported conflicting data, deformability of mature resting neutrophils appears to be similar in healthy preterm neonates to their full-term counterparts and adults.49 However, the release of immature neutrophils from the NSP during sepsis is associated with an overall reduction in neutrophil deformability.50
Interventions such as administration of recombinant G-CSF increases the expression of β2-integrins, but lowers L-selectin expression on neonatal neutrophils (both term and preterm).51 In contrast, early dexamethasone administration decreases β2 integrin expression on neutrophils.52
Chemotaxis.
Chemotaxis is defined as the directed cellular migration along the concentration gradient of a chemoattracting substance (see Figure 54-1). This movement involves a series of orchestrated events, including the binding of the chemoattractant to cell surface receptors, generation of an intracellular second messenger that is coupled to the receptor-ligand binding, and remodeling of the plasma membrane and cytoskeleton to produce shape changes and proper orientation of the cellular contents toward the highest concentration of the chemoattractant. Morphologically, the PMN orients toward the chemoattractant with the formation of a lamellipodium along the leading edge. Most of the intracellular organelles remain at the posterior pole of the cell (uropod). As the cell moves, the leading edge adheres to available surfaces, and contraction of cytoskeletal microfilaments (actin and myosin) pulls along the rest of the cell. Many aspects of this process are poorly developed in PMNs isolated from neonatal blood. Some of the chemotactic defects of PMNs present during the neonatal period persist throughout early childhood.
Neutrophils from both term and preterm neonates have an impaired chemotactic response and migrate at only about half the speed traveled by adult cells.53,54 Although neutrophils from term infants achieve normal chemotactic function by 2 weeks after birth, such postnatal neutrophil maturation begins 2 to 3 weeks after birth in immature preterm infants and proceeds very slowly.55 Neutrophils from preterm infants born at 34 to 36 weeks’ gestation achieve normal chemotaxis by 40 to 42 weeks’ postconceptional age (PCA). In more immature preterm infants (<34 weeks), neutrophil chemotaxis improves with time but remains impaired in comparison to adults even at 42 weeks’ PCA.56 The presence of various clinical confounders makes it difficult to separate the effects of clinical stress from the effects of prematurity on neutrophil function. Gram-negative sepsis may depress neutrophil chemotaxis whereas superficial infections are associated with enhanced chemotaxis.57,58
Neonatal neutrophils bind various chemoattractants normally. However, chemoattractant-induced membrane depolarization, calcium transport, and sugar uptake are relatively less efficient. Neonatal neutrophils show an incremental chemotactic response to increasing chemokine concentrations, but these responses remain lower than adult neutrophils.59 The chemotactic defect in neonatal neutrophils may be multifactorial, affected by factors such as a larger, poorly motile neutrophil subpopulation, impaired calcium mobilization, and aberrations in intracellular signaling pathways such as NF-κB activation.60,61 Lower Mac-1 expression can also impede chemotaxis due to impaired neutrophil interaction with the extracellular matrix.60,62
In preterm infants, intrapartum exposure to magnesium sulfate reduces both neutrophil chemotaxis and random motility.63 Theophylline concentrations in the high therapeutic range (84 µmol/L or 15 µg/mL) cause dose-dependent reductions in neutrophil chemotaxis. Cells from preterm infants are particularly sensitive to this effect. In contrast, theophylline concentrations in the low therapeutic range (28 µmol/L or 5 µg/mL) increase neutrophil activity.64 Indomethacin, too, has an adverse effect on neutrophil chemotaxis, which is more pronounced in preterm infants.65 Both G-CSF and GM-CSF increase neutrophil chemotactic responsiveness to other chemoattractants.66
Phagocytosis.
Phagocytosis is a process of particle ingestion. Most particulate matter must be opsonized (coated) with IgG, complement fragments C3b or iC3b, fibronectin, or other proteins before being recognized and engulfed by PMNs (Figure 54-3). Neutrophils express IgG receptors such as Fcγ receptors I to III (or CD16, CD32, CD64), C3b (CR1), and iC3b (CR3).42 After binding of the opsonized microbe by an appropriate cell surface receptor, the PMN extends pseudopods to surround the particle and form a phagocytic vacuole. Microbes also may be ingested without opsonization through several interactions such as lectin-carbohydrates on bacterial fimbriae that interact with neutrophil glycoproteins, protein-protein (such as filamentous hemagglutinin that express the argly-asp), and hydrophobic-protein (bacterial glycolipids and neutrophil integrins) interactions.67

Neutrophils from preterm neonates show impaired phagocytosis, which corrects by the late third trimester or term gestation to become comparable to that of adults.54 Preterm neutrophils ingest particles more slowly and ingest fewer bacteria. The lack of opsonic activity is an important consideration because preterm infants often have lower concentrations of specific antibodies. Adult neutrophils lose their phagocytic efficiency if suspended in serum of preterm infants.68 Similarly, neutrophils of preterm neonates can increase their phagocytic function following exposure to adult serum or therapeutic immunoglobulin preparations.68–70
Compared with term neonates and adults, preterm neutrophils have a lower expression of CD16 (FcγRIII) and CD32 (FcγRII), the two most abundant neutrophil IgG receptors. In “stressed” preterm neonates with severe RDS or sepsis, CD16 expression may be even lower.71 Whereas CD16 expression normally increases to adult levels over the first 3 weeks of life, CD32 deficiency does not correct with time. CD32 is the high-affinity receptor for IgG2 (important against encapsulated bacteria), and hence CD32 deficiency may represent an important immune defect in preterm neonates.72 Unlike CD16 and CD32, CD64 expression on neonatal neutrophils (both preterm and term) may be higher than neutrophils from adult subjects.73 CD64 is not affected by neonatal “stress,” as in respiratory distress or prolonged rupture of membranes, and data suggest that CD64 might be a useful early marker for bacterial infections.74
Recombinant G-CSF and GM-CSF both activate neutrophil phagocytosis. The benefits of intravenous immune globulin (IVIG) as a source of opsonic activity remain uncertain.68,70,75 A major limitation may be in the formulation of current IVIG preparations, which may not have adequate concentrations of antibodies against neonatal pathogens.76
Microbicidal Activity.
The phagolysosome provides an enclosed space in which an ingested microbe is exposed to high concentrations of toxic substances, while limiting the exposure of the phagocyte and other cells to these potentially injurious agents.77 The major killing mechanism in neutrophils involves the generation of highly reactive free oxygen radicals in a “respiratory burst.” An NADPH-dependent respiratory burst oxidase localized on the cell membrane (and therefore the phagosome membrane) reduces molecular oxygen (O2) to superoxide anion (O•2−).78 Subsequent generation of hydrogen peroxide (H2O2) and the hydroxyl radical (OH•; formed in the presence of iron) also contributes to the microbicidal capacity of neutrophils.79
These oxygen-dependent bactericidal mechanisms can be broadly divided into myeloperoxidase (MPO)-independent (such as hydrogen peroxide) and MPO-dependent (MPO catalyzes reactions between H2O2 and halides to form highly reactive products).80 H2O2 is a weak bactericidal agent per se, but the MPO-H2O2-halide system increases its efficacy by nearly 50-fold. The bactericidal effects of free oxygen radicals are caused by oxidizing effects on various components of the bacterial cell wall.79
Neutrophils also have elaborate nonoxidative killing mechanisms such as low pH (as low as 6.0), defensins, bactericidal/permeability-increasing protein (BPI), lactoferrin, lysozyme, and a variety of cationic proteins. Defensins are broad-spectrum antimicrobial peptides with activity against Gram-positive and Gram-negative bacteria, fungi, and enveloped viruses.81 Bactericidal/permeability-increasing protein binds lipopolysaccharide (LPS) and blocks its effects, can damage the outer membrane of Gram-negative bacteria, and has some opsonic activity.82 Lactoferrin, an iron chelator, is bacteriostatic because it deprives bacteria of the iron required for growth. Lactoferrin is also involved in neutrophil degranulation, oxygen radical production, and granulocytopoiesis. Lysozyme hydrolyzes a glycoside bond in the bacterial cell wall peptidoglycan. Primary granules also contain other cationic antibacterial proteins such as azurocidin, indolicin and cathelicidins.77,83
Although preterm neutrophils have a higher oxygen consumption and normal/elevated release of superoxide and H2O2, the respiratory burst is, overall, depressed. This deficiency is more marked in preterm infants with a high severity of sickness.54,84,85 Neutrophil oxidative burst remains impaired in preterm infants despite opsonization with IgG and complement.86
Perinatal events can influence the respiratory burst in neonatal neutrophils. Labor and vaginal delivery activate the generation of free oxygen radicals in neonatal neutrophils. In contrast, perinatal distress can suppress the neutrophil respiratory burst. Both LPS and cytokines such as interferons and TNF can prime neutrophils for an accelerated respiratory burst in vitro, but prolonged exposure to these agents (during sepsis) can dampen their effect. Reduced respiratory burst activity in preterm infants correlates with impaired intracellular killing of S. aureus or E. coli. Whereas bacterial killing by neutrophils from term neonates has consistently been found to be normal, killing of staphylococci was impaired in preterm neonates with birth weight less than 2000 g.87
The postnatal maturation of respiratory burst response varies according to gestational age. During the first week, infants born at 24 to 28 weeks have a lower respiratory burst than those born at 29 to 35 weeks. The differences between preterm infants born at different gestational ages disappear in about 2 months, but overall, neutrophils from preterm infants continue to have a weaker oxidative burst than adults. The postnatal maturation of the respiratory burst may not be seen at all in sick preterm infants receiving intensive care.88
In “stressed” preterm infants receiving intensive care, recombinant GM-CSF can boost the neutrophil respiratory burst to levels seen in term neonates.89 Similarly, G-CSF can enhance neutrophil respiratory burst response in septic preterm infants.90,91 In adults, hypertonic saline may enhance host response to bacterial challenge by augmenting superoxide formation in neutrophils.92 Intracellular killing may also be augmented by fluoroquinolones such as ciprofloxacin, which have a potent intraphagosomal bactericidal activity against both Gram-positive and Gram-negative bacteria.93
Degranulation.
Neutrophils contain two major types of granules83: (1) the “azurophilic” granules (stain positive with the azure A dye) and (2) “specific” granules (do not stain with azure A). Azurophilic granules contain myeloperoxidase (MPO), proteolytic enzymes such as cathepsins, proteinase-3, and elastase, and antimicrobial proteins such as defensins and the bactericidal permeability-increasing protein (BPI). These granules release their contents into the phagolysosomes and are involved in intracellular killing. The specific granules contain antibacterial agents such as lactoferrin and lysozyme, receptors for complement components, and bacterial products such as f-MLP. Specific granules fuse with the cell membrane to release their contents by exocytosis, and also bring functionally important membrane proteins such as integrins, cytochrome-b558, and receptors for chemotactic agents and opsonins to the cell surface. Specific granules play an important role in extracellular killing.94
Neutrophils from term neonates have granule contents and degranulation responses similar to adults.95 However, neutrophils from preterm infants have a lower capacity to release BPI, elastase, and lactoferrin than do those in adults and term infants.54,96 Granulocyte colony-stimulating factor activates mature neutrophils without degranulation of primary granules,97 whereas GM-CSF induces degranulation and exocytosis of granule contents.98 On the other hand, anti-inflammatory agents such as corticosteroids and indomethacin inhibit the degranulation of secondary granules.99
Summary.
Polymorphonuclear neutrophils of term and preterm infants are limited in chemotactic, phagocytic, and microbicidal activities.
The physiologic rationale for the use of hematopoietic growth factors to improve neutrophil function qualitatively and quantitatively has been well established. The use of G-CSF and GM-CSF has been shown to increase the neutrophil storage pool, induce neutrophilia, and improve many neutrophil functions.7,9,100–108 In meta-analysis, G-CSF administration was shown to reduce mortality in neonates with sepsis.109 However, when the nonrandomized studies were excluded, the analysis did not remain statistically adequate. Granulocyte colony–stimulating factor therapy is generally well tolerated in neonates, and many centers now use recombinant G-CSF in infants with severe neutropenia (absolute neutrophil counts <500 for 48 hours or between 500 and 1000 for more than 5 to 7 days).110 However, based on current literature, a clear recommendation for the use of these growth factors to decrease the incidence of morbidity and mortality associated with sepsis in newborns cannot yet be made.111 Further studies are needed in high-risk neonatal subgroups with sepsis, as preliminary evidence suggests that such an approach may be more effective. The newer, longer-acting polyethylene glycol-conjugated or glycosylated forms of G-CSF also hold promise as viable therapeutic alternatives.
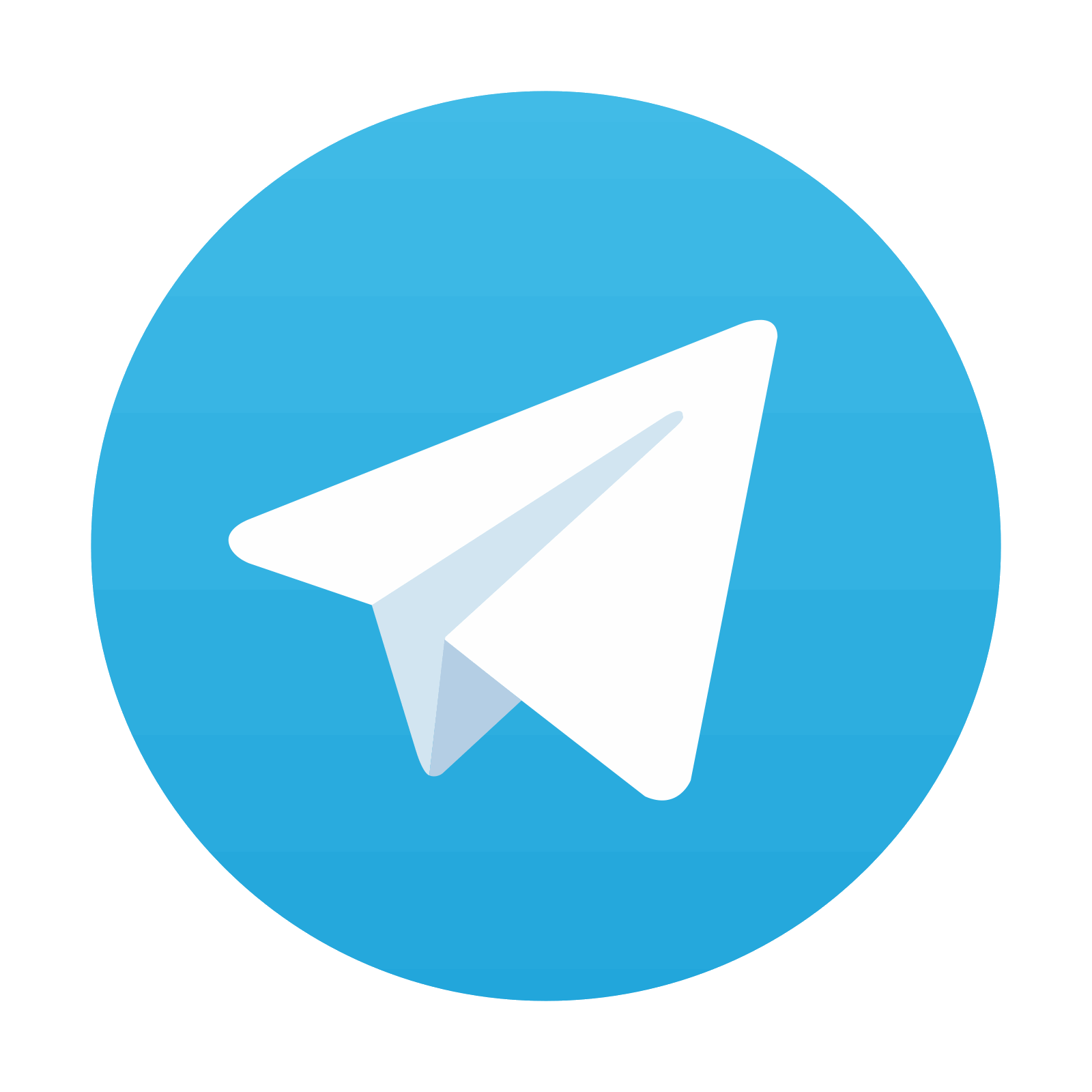
Stay updated, free articles. Join our Telegram channel
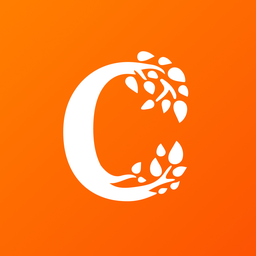
Full access? Get Clinical Tree
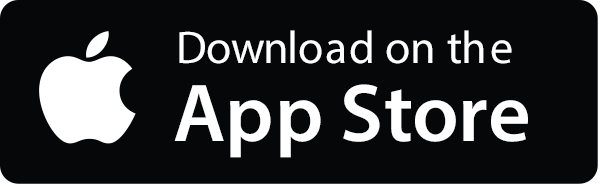
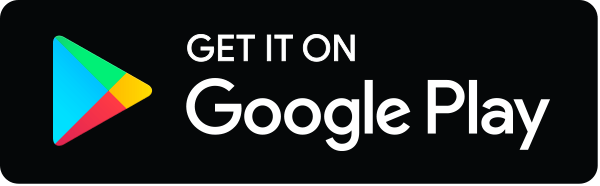