INTRODUCTION
To effectively incorporate radiation treatment into cancer care, clinicians must understand the fundamental concepts and vocabulary used in radiation oncology. Clinically, radiation therapy, often combined with chemotherapy, can be used as primary treatment for many gynecologic malignancies (Table 28-1). Additionally, radiation therapy may be recommended postoperatively if the probability of tumor recurrence is high. Radiation therapy is also used frequently in the relief of symptoms caused by metastasis of any gynecologic cancer.
RADIATION PHYSICS
Radiation therapy is the focused delivery of energy in tissue to accomplish controlled biologic damage. Radiation used in this therapy can occur as electromagnetic waves or particles.
Photons (x-rays) and gamma rays are the two types of electromagnetic radiation used for radiation therapy. Photons, used in external beam therapy, are produced when a stream of electrons collides with a high atomic number target (tungsten) located in the head of a linear accelerator (Fig. 28-1). In contrast, gamma rays originate from unstable atom nuclei and are emitted during decay of radioactive materials, also termed radionuclides, which are widely used in brachytherapy.
FIGURE 28-1
Block diagram of a linear accelerator used to create external beam radiation. Either photon beams or electron beams may be produced. A. Photon beam therapy is suited for deep tumors such as the cervical cancer shown here. Beam energy is measured in million volts (MV). B. Electron beam therapy is indicated for superficial lesions such as inguinal lymph nodes. Beam energy is measured in million electron volts (MeV).
Whereas electromagnetic waves are defined by their wavelengths, particles are defined by their masses. For clinical use, particles include electrons, neutrons, protons, helium ions, heavy charged ions, and pi mesons. Except for electrons, which are available in all modern radiation oncology centers, and protons, other particles have limited clinical use. Proton facility numbers are expanding, with 14 facilities operating in the United States and 10 additional centers under construction.
Particles are produced by linear accelerators or other high-energy generators and are usually delivered by external beam. Of clinically used particles, electrons are negatively charged and deposit most of their energy near the surface. In contrast, heavy charged particles, such as protons, deposit most of their energy in the absorbing tissues as their velocity decreases, that is, near the end of the particle path (the Bragg peak effect). Because of this, a major advantage of proton therapy is the lack of an exiting dose through normal tissues. Proton therapy use for gynecologic malignancies is primarily investigational. But as an example, proton therapy can treat affected deep pelvic and paraaortic lymph nodes, while sparing unnecessary dosing and injury to anterolateral organs, such as the bowel and kidneys (Fig. 28-2).
FIGURE 28-2
Proton dose distribution in a patient with cervical cancer receiving treatment to the paraaortic lymph nodes. The proton beam originates posteriorly and is directed anteriorly. The red arrow shows the target volume (dark magenta outline). The area anterior to this is bowel (orange outline), which is significantly spared from radiation.
Also called radioisotopes, radionuclides undergo nuclear decay and can emit: (1) positively charged alpha particles, (2) negatively charged beta particles (electrons), and (3) gamma rays. Radionuclides commercially available are shown in Table 28-2. Cesium and iridium are commonly used in gynecologic brachytherapy.
Element | Radiation Energy (MeV) | Half-Life | Clinical Use |
Cesium-137 | 0.6 | 30 years | Brachytherapy |
Iridium-192 | 0.4 | 74 days | Brachytherapy |
Cobalt-60 | 1.2 | 5 years | Brachytherapy |
Iodine-125 | 0.028 | 60 days | Brachytherapy |
Phosphorus-32 | 1.7 | 14 days | Intraperitoneal instillation |
Gold-196 | 0.4 | 2.7 days | Intraperitoneal instillation |
Strontium-89 | 1.4 | 51 days | Diffuse bone metastasis |
One of the main types of radiation-producing units is the linear accelerator, also called a linac. A linac can produce both photon and electron beams (see Fig. 28-1). In the photon-therapy mode, indicated for deep-seated tumors, the accelerated electron beam is guided to hit a metal target to produce photons. The unit used to describe the energy of a photon beam is MV (million volts). In the electron-therapy mode, indicated for superficial lesions, the electron beam strikes a lead scattering foil instead of the metal target. The unit for electron beam energy is expressed in MeV (million electron volts). Figure 28-3 displays a linac with three moveable components: gantry, treatment head, and couch. These components can all rotate 360 degrees, which allows multiple fields and angles to achieve optimal dose delivery to a tumor.
When electromagnetic radiation is used in daily clinical practice, it contacts target tissues, and energy is transferred to those tissues. This transfer creates ions by dislodging electrons from atoms within these tissues. These electrons then collide with surrounding molecules to initiate radiation damage.
There are three mechanisms involved in energy transfer: (1) photoelectric effect, (2) Compton effect, and (3) pair production (Fig. 28-4). Depending on the energy level of the incident radiation, one of these mechanisms will predominate. The photoelectric effect is dominant if the incident energy is low (less than 100 kV). The Compton effect dominates in mid- to high energy ranges (1 MV to 20 MV) and is the most important in clinical radiation therapy. Last, pair production occurs when a photon beam with very high energy (beyond 20 MV) strikes the electromagnetic field of the nucleus.
FIGURE 28-4
When electromagnetic radiation impacts target tissues, energy is transferred to those tissues. The three mechanisms involved in this energy transfer are the photoelectric effect, Compton effect, and pair production. Both photoelectric effect (A) and Compton effect (B) result in creation of fast electrons, which then initiate the biologic process of radiation damage. A. With photoelectric effect, radiation interacts with an inner orbital electron. B. With Compton effect, interaction occurs with an outer orbital electron. C. During pair production, impact of radiation on the atom’s nuclear forces produces a positron-electron pair. When a positron later combines with a free electron in these tissues, two photons are created, which can then lead to radiation damage.
Controlled biologic damage is accomplished by greater selective radiation dose distribution within malignant tissue than within the surrounding, “innocent bystander” normal tissues. This is achieved by using radiation beams with differing physical properties to define the spatial distribution of an absorbed dose when these beams strike tissue. Ideally, an absorbed radiation dose is as conformal as possible. Perfect conformality is achieved when the targeted malignant tissue absorbs 100 percent of the prescribed dose and the adjacent normal tissues absorb 0 percent. In practice, this cannot be obtained. However, both acute radiation side effects and the potential for late, delayed radiation complications can be minimized as the spatial radiation dose distribution approaches this ideal.
A depth-dose curve specifically illustrates the dose distribution of a given radiation beam as it penetrates tissues. Radiation oncologists rely on these curves when choosing a radiation beam with an appropriate energy to reach a given tumor. With electron beam therapy, the maximum dose lies close to the surface, and therefore, electron beam therapy is indicated for targets that are close to the skin surface, such as metastatic cancer to the inguinal lymph nodes. With high-energy photons, the maximum dose is deposited well below the surface. Beyond this point, the dose gradually tapers as energy is absorbed by the deep surrounding tissues. This explains the so called skin-sparing effect of high-energy photons. A patient with a pelvic malignancy is usually treated with at least 6-MV photon beams.
This is the discipline of calculating the radiation dose absorbed by the patient. Dosimetric calculations are based on the depth-dose measurements of the radiation beams used to treat an actual patient. The dose distribution is usually displayed as a colorful map overlaid on the radiologic images of the patient. These calculations predict the absorbed dose in a given situation.
Quantification of the absorbed radiation dose is essential as it correlates with the biologic effect of radiation. The current Standard International unit for absorbed dose is gray (Gy). One Gy equals 100 rad or 1 joule/kg. Clinically, the radiation doses for curative and palliative treatment are 70 to 85 Gy and 30 to 40 Gy, respectively.
RADIATION BIOLOGY
The DNA molecule is the target for the biologic effect of radiation on mammalian cells. DNA injuries involve its strands, bases, and cross-links, but the hallmark damage is breaking of single- and most importantly double-stranded DNA. Double-strand breaks lead to DNA fragmentation when two or more breaks are formed and when cells attempt to repair these strand breaks. The DNA pieces may rejoin incorrectly, leading to gene translocation, mutation, or amplification and, ultimately, cell death.
Radiation can interact directly or indirectly with the atoms in the DNA molecule. Direct actions create ions that then initiate the biologic damage process. This direct effect is predominant with high linear energy transfer (LET) particles such as protons, fast neutrons, and heavy ions (Fig. 28-5). However, most DNA damage is caused indirectly, which is the predominant effect with low-LET particles such as photons. Indirect DNA damage occurs through an important chemical intermediate, the hydroxyl radical (OH·), which is highly reactive because of its unpaired electron.
The two main cell death pathways after radiation are apoptosis and mitotic catastrophe. Mitotic catastrophe is thought to be the most common mechanism of cell death after radiation exposure. With this, cells with damaged DNA enter mitosis prematurely, before DNA can be repaired, and die attempting to complete the next two to three mitotic cycles. Apoptosis, or programmed cell death, occurs after an intracellular stress, such as radiation-induced irreparable double-strand breaks. A series of events develop rapidly within hours, leading to cell membrane blebbing, apoptotic body formation in the cytoplasm, chromatin condensation, nuclear fragmentation, and DNA laddering (Okada, 2004).
In classic radiation biology, there are four mechanisms by which cells respond to radiation. Cellular repair can be described by sublethal damage repair (SLDR) and potentially lethal damage repair (PLDR). SLDR occurs when a radiation dose is split into two or more fractions and a few hours separate the fractions. Cells have time to repair their damage, and their survival rate increases. The last process seen in SLDR is repopulation, which is the tissue’s response to replenish the cell pool (Trott, 1999).
Following the initial repair of sublethal damage, reassortment begins. Within a tumor, proliferating cells are in different phases of the cell cycle (Fig. 27-1). Cells in mitosis (M) and G2 are most sensitive to radiation. Conversely, cells in G1 and S (DNA synthesis) phases are less sensitive (Pawlik, 2004). When exposed to radiation, those cells that are in the G2/M phase are killed. During reassortment, surviving cell populations restart their progression through the mitotic cycle.
The fourth “R” of radiation biology theory is reoxygenation. A tumor cell population is composed of oxygenated and hypoxic components. Cells located within 100 microns of blood capillaries are oxygenated, and beyond 100 microns, cells are hypoxic. After radiation, the oxygenated cells are killed by chemical intermediates described earlier. Following cell death, the tumor shrinks and allows hypoxic cells to be positioned within the oxygen diffusion range of blood capillaries and become oxygenated.
For low-LET radiation, the linear-quadratic curve has been adopted to explain the relationship between the fraction of cells surviving a given dose of radiation (Fig. 28-6). The initial linear (alpha) portion of the curve shows that the probability of cell death is proportional to the radiation dose. In the higher-dose region, the curved quadratic (beta) portion indicates that the probability of cell death is proportional to the square of the dose.
FIGURE 28-6
Linear quadratic mammalian cell survival curve. The cell survival is plotted on a logarithmic scale. The dose (in Gy) is on a linear scale. The typical cell survival curves with low-LET (linear energy transfer) (blue curve) and with high-LET radiation (red line) are shown. With low-LET x-ray doses, the alpha (linear) portion of the curve is flat and shows that cell survival is proportional to the dose. However, as the dose increases, the beta (quadratic) portion bends, which implies that cell survival is proportional to the dose squared. In contrast, with high-LET radiation, such as neutrons, the survival curve is straight.
The alpha/beta ratio reflects the response of normal tissues to radiation. Early-responding tissues have a high alpha/beta ratio and will manifest reactions to radiation within a few days to weeks after treatment. Examples are tissues with high proliferation rates such as bone marrow, reproductive organs, and gastrointestinal tract mucosa. Preventatively, by administering multiple small radiation dose fractions, there is more sublethal damage repair, and early acute reactions can be decreased.
In contrast, late-responding tissues show clinical reactions only weeks to months after completion of a radiation therapy course. Examples are the lung, kidney, spinal cord, and brain. These tissues have a low alpha/beta ratio and are slow to respond. More time is needed to repair sublethal damage, and thus high-dose per fraction radiation therapy can easily lead to severe late complications.
RADIATION ONCOLOGY PRACTICE
The expertise of the radiation oncologist who is designing and monitoring a course of radiation treatment is paramount. Radiation oncology is as inherently “operator dependent” as surgical oncology. The radiation oncologist carves out, en-bloc, a volume of cancerous tissue, its local extensions, and its regional lymphatics similar to radical surgical resection, while accommodating the patient’s comorbidities, general health status, and surrounding normal tissue quality.
As cancer management is frequently multimodal, superior treatment outcomes are highly dependent on clear communication between radiation oncologists and their colleagues in surgical and medical oncology. Optimal integration of diagnostic imaging and histologic evaluation is also vital to planning and implementing potentially curative radiation therapy. As such, early involvement of the radiation oncologist in patient evaluation and counseling enhances the probability of effective and coordinated cancer care.
Parameters that affect the efficacy and safety of a radiation course include the total radiation dose applied, the size of each radiation “fraction” (treatment), the time between treatments (“fractionation schedule”), and the elapsed time to deliver the total prescribed dose.
Successful eradication of a localized cancer requires that the cancer cells be killed more rapidly and efficiently than surviving cancer cells can proliferate and repopulate. Because of the inevitable exposure of some normal tissues to substantial radiation, there are limits to the total radiation dose that can be prudently administered to a given target volume. In general, delivery of this dose over the shortest feasible elapsed time will maximize efficacy. Accomplishing this objective is limited by the known deleterious consequences of a high dose per fraction on normal tissues. As noted, this can result in delayed radiation injury expressed months or years after therapy completion. For this reason, radiation delivered with curative intent is generally administered in daily treatments (Monday through Friday) of 1.8 Gy to 2.0 Gy. Cumulative doses range from 45 Gy to treat microscopic disease to 70 Gy or more to treat gross disease. This concern for delayed injury is lessened when short courses of radiation are administered with palliative intent, often in dose fractions of 2.5 Gy to 4.0 Gy.
Regimens involving treatments given more than once a day are reserved for selected cases. In such instances, increased local tumor control and decreased long-term complications may be achieved by manipulating both fraction size and overall treatment time. This manipulation leads to a variety of altered fractionations. Two major strategies have been employed, namely, hyperfractionation and accelerated treatment.
With hyperfractionation, the reduction of late damage to normal tissues is sought, and accordingly, a smaller dose per fraction is given. Two or more fractions are administered each day, typically 6 hours apart to provide an interval for normal tissue repair.
Accelerated fractionation entails shortening the treatment duration with or without a decrease in total dose to overcome tumor cell repopulation. The usual weekend break is either shortened or eliminated. With accelerated treatment, however, severe acute reactions are frequent.
Altered fractionation has been studied in gynecologic cancers. Radiation Therapy Oncology Group (RTOG) trials 88-05 and 92-10 were Phase II trials that investigated the use of twice-daily radiation and chemoradiation, respectively, in advanced cervical cancer. RTOG 88-05 showed similar local control, survival rates, and toxicity compared with historical controls. However, when chemotherapy and larger-field twice-daily radiation were added, there were unacceptable rates of late Grade 4 toxicity (Grigsby, 2001, 2002).
External beam radiation therapy is indicated when an area to be irradiated is large. For example, the fields needed to treat a locally advanced cervical cancer may cover the whole pelvis and occasionally, the paraaortic lymph nodes.
Conformal radiation therapy (CRT) describes a radiation treatment technique that maximizes tumor damage while minimizing injury to the surrounding normal tissues. To this goal, the radiation oncologist must know the precise extent of the cancer to be irradiated and its relationship to surrounding normal tissues. This process begins with a review of the patient’s cancer imaging including computed tomography (CT), magnetic resonance (MR) imaging, and positron emission tomography (PET). Imaging, along with careful review of the patient’s pathology and operative report, assists in defining the 3-dimensional (3-D) target (tumor or regions of potential microscopic tumor spread) and normal tissue volumes.
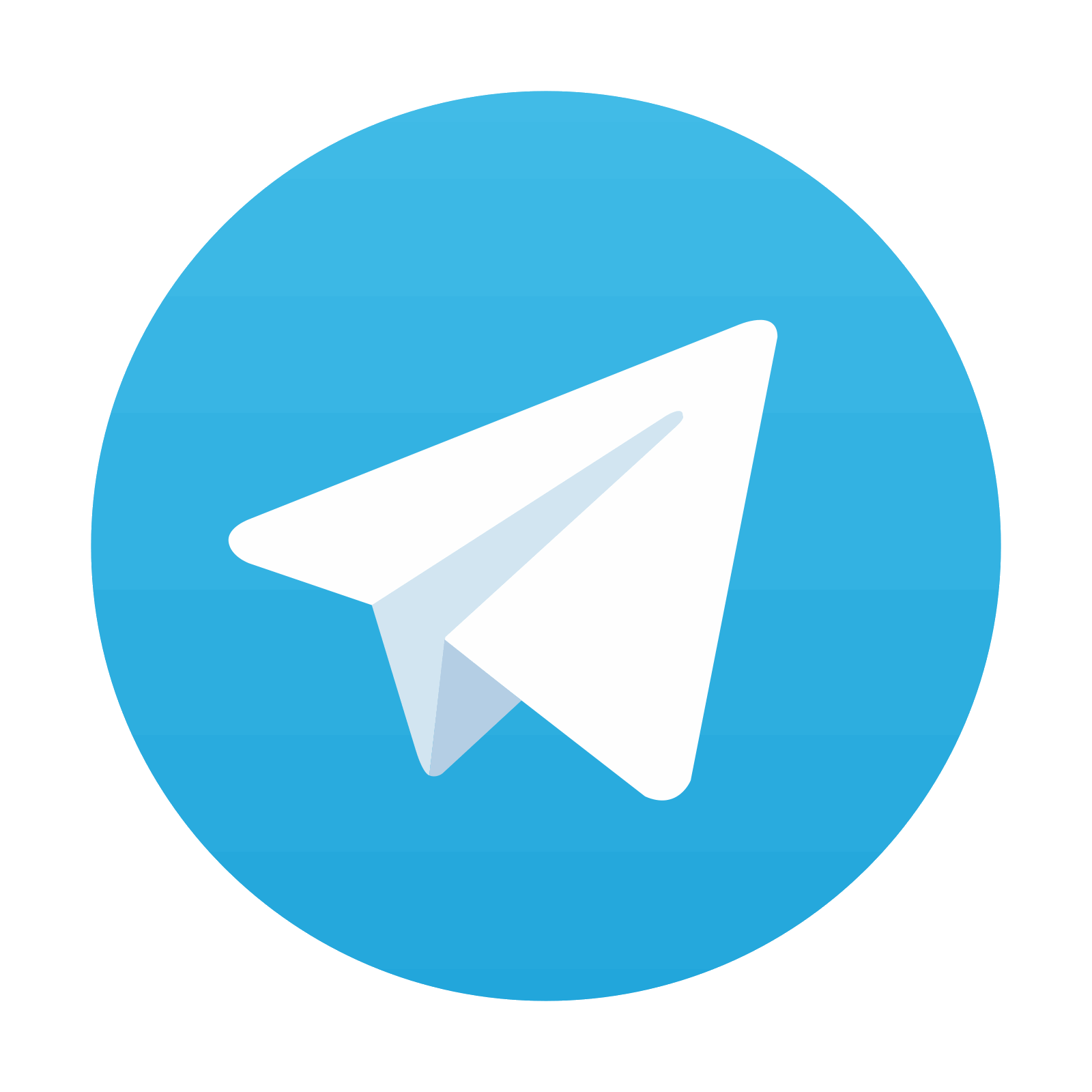
Stay updated, free articles. Join our Telegram channel
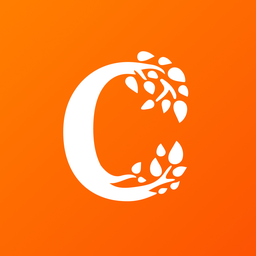
Full access? Get Clinical Tree
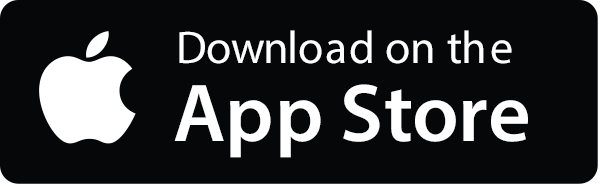
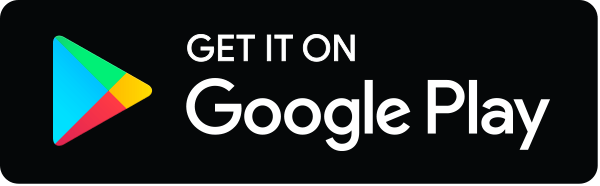