Calcium and phosphate homeostasis during pregnancy and lactation
Pregnancy
Pregnancy increases the requirement for many nutrients, including minerals. If exogenous sources are insufficient, the mother’s system will be called upon to satisfy the demands. In particular, the maternal calcium storage found in bone mass is available, and therefore potentially vulnerable.
Calcium
Approximately 50% of the total serum calcium is protein bound, and 50% is a free (unbound) ionized fraction. The binding is predominantly to albumin, some to immunoglobulins, and a small fraction to phosphate, citrate, sulfate or other anions. Early in gestation, there is a fall in the total serum calcium which is not considered to be metabolically important, because it is a consequence of a decreased serum albumin level due to hemodilution. The biologic activity of calcium relates to its free (unbound) ionized fraction which remains constant throughout pregnancy [1]. Since the binding is largely to albumin, variations in albumin concentration will alter the total serum calcium level and could lead to misinterpretation unless corrective calculations are performed. In general, the plasma total calcium concentration falls by 0.8 mg/dL (0.02 mmol/L) for every 1.0 g/dL (1 g/L) fall in the plasma concentration of albumin. The formula to determine the corrected total calcium level in the context of a low albumin that is often used is as follows:
Corrected calcium in conventional (US) units, i.e. mg/dL = measured total calcium in mg/dL + (0.8 × (4.0 − [albumin in g/dL]))
Corrected calcium in SI units, i.e. mmol/L = measured total calcium in mmol/L + (0.02 × (40 − albumin measured in g/L))
However, these calculations give only a rough estimate of the actual free or ionized calcium, which is the main concern. Since most clinical laboratories now measure ionized calcium readily, it is recommended that ionized calcium be measured directly whenever there is a question of whether a pregnant patient has an abnormal calcium rather than relying on the estimations provided by these or similar formulas.
The total body calcium content is 1000–2000 g, and 99% of the calcium in the body of adult individuals is in the skeleton. About 200–400 mg of calcium are absorbed daily by active transport from the proximal small bowel in the nonpregnant adult. During pregnancy, however, intestinal absorption increases twofold, particularly during the third trimester [2–4]. Increased intestinal calcium absorption is already present at 12 weeks, which is the earliest gestational age that has been studied. It is associated with higher serum levels of 1-alpha-25-dihydroxyvitamin D (1α,25-(OH)2D3) as well as increased intestinal expression of the vitamin D-dependent calcium-binding protein calbindin-9K. Prolactin (PRL) and human placental lactogen (HPL) are believed to be other factors contributing to the increased intestinal calcium absorption. Because the increased intestinal calcium absorption starts so early in gestation and well before the peak demands of the third trimester, it is thought that some calcium is stored in the maternal skeleton in anticipation for subsequent demands. The fetus accumulates about 30 g of calcium and 16 g of phosphorus in its own tissues; 80% of the total fetal calcium accumulation takes place in the third trimester, which is when fetal skeletal growth is maximal. At that time, 250–300 mg of calcium are deposited daily into the fetal skeleton. Without an anticipatory rise in calcium absorption, the increased needs would have to be drawn from the maternal skeleton, and some of it probably is. Current research indicates that the major adaptation to the increased calcium demands during pregnancy is the higher intestinal calcium absorption with, perhaps, some contribution from the maternal skeleton [5].
The daily dietary calcium requirement during pregnancy is 1200 mg, which is 400 mg higher than outside pregnancy [6]. Some women do increase their dietary calcium during pregnancy [7], but there is concern that the calcium intake of most women may be less than the recommended 1200 mg/day [6,8].
Under normal circumstances, 98% of calcium filtered by the kidney is reabsorbed, mostly in the proximal tubules. However, in pregnancy the urinary calcium excretion is higher than outside pregnancy and correlates with the increased glomerular filtration rate (GFR) [9]. The increased urinary calcium excretion has also been detected as early as 12 weeks of gestation. Besides the higher GFR of pregnancy, the increased intestinal calcium absorption and the higher calcitonin (CT) levels of pregnancy are believed to be contributing factors favoring renal calcium excretion.
Early in gestation fetal serum calcium concentration is lower than the maternal serum calcium [10] but at term, fetal levels exceed the maternal for both total [10,11] and free ionized calcium [12]. The fetal calcium concentration increases from 5.5 mg/dL (1.37 mmol/L) in early pregnancy to 11 mg/dL (2.75 mmol/L) at term. Therefore calcium is transported across the placenta against a gradient indicating the existence of an active transport mechanism [13]. At present, a parathyroid hormone-related protein (PTHrP) is considered to be the most likely candidate for this role [14]. It may be of placental origin [15] and some may be produced in the mammary glands [16], but the exact source or sources of this peptide or in what proportion each contributes to the maternal circulation have not been conclusively established [17] (see PTHrP section below).
Phosphorus
Of the approximately 600 g of total body phosphorus, 85% is in the skeleton and the remainder is a major intracellular component. In contrast to calcium, about 88% of inorganic phosphate is free and not protein bound. The normal levels in adults are 2.5–4.5 mg/dL (0.81–1.45 mmol/L). Phosphate concentration is labile and may change rapidly by as much as 50% on a given day. There is a normal daily variation with a nadir between 7 and 10 am. Food intake also has a major effect and after eating, plasma phosphate falls because it enters the cells, along with glucose, under the influence of insulin. Therefore, samples for phosphate estimation should be taken early in the morning with the patient fasting.
Phosphate is abundant in normal foods. Its absorption is very efficient, 65% by the small intestine, and it may increase to 85–90% through stimulation by 1α,25-(OH)2D3. Dietary deprivation, short of actual starvation, does not lead to deficiency. Nevertheless, nonabsorbable antacids, if taken in large amounts, can interfere with phosphate absorption by binding it in the intestinal lumen. Under normal circumstances, the daily requirement of 500–1000 mg is easily satisfied by gastrointestinal absorption.
The kidney plays a major role in regulating phosphate balance. After glomerular filtration, 80–90% of phosphate is reabsorbed in the proximal tubules. A rise in plasma phosphate is countered by increased renal excretion. Bone uptake and release of phosphate also contribute to homeostasis, but the renal mechanism predominates.
Inorganic phosphorus economy in pregnancy is based upon an increase in its renal tubular reabsorption, and also by enhanced intestinal absorption mediated by 1α,25-(OH)2D3 [18]. This is important as inorganic phosphorus, no less than calcium, is essential for the growing fetus.
Vitamin D
The healing effect of ultraviolet light on bone lesions caused by rickets was recognized in 1919. Soon afterwards, it was demonstrated that sunlight activates a sterol in the skin that was labeled the “antirachitic agent.” This process is the photochemical conversion of 7-dehydrocholesterol to cholecalciferol or vitamin D3 after the skin is exposed to ultraviolet radiation. Although the diet also contributes to the body stores of vitamin D, it does so to a lesser extent and about 90% of circulating vitamin D is skin derived [19]. Absorption from the proximal small intestine is aided by bile salts as it is for other fat-soluble vitamins. Natural dietary sources include fish oils, eggs, and liver. Fortified cereals and dairy products are increasingly being relied upon. Animal sources provide vitamin D3 while plant sources yield vitamin D2 (ergocalciferol). Vitamin D2 is 30% less effective than vitamin D3 in maintaining adequate serum 25-hydroxyvitamin D (25-OHD) levels, and therefore larger doses will have to be administered when vitamin D2 is used instead of vitamin D3 [20,21].
More recently, there has been more reliance on dietary sources due to overall reduced sun exposure as modern lifestyle changes lead to less time spent outdoors, widespread use of sun-blocking creams to reduce the risk of skin cancer, dress style, and residing in latitudes with reduced sunlight. Studies from many countries are reporting a high prevalence of vitamin D deficiency not only in the general population but also in women of childbearing age during pregnancy and lactation [22–24]. There is also a growing consensus that current vitamin D intake is insufficient in populations with reduced exposure to sunlight and increasing concern not only about calcium and bone metabolism but also about preventing other diseases. Interest is accumulating in the many extraskeletal actions of vitamin D, including immune maintenance which might help to prevent autoimmune disorders (multiple sclerosis, systemic lupus erythematosus, rheumatoid arthritis, etc.), certain cancers (breast, colon, ovarian), type 2 diabetes, asthma, and several other chronic conditions as well. Paradoxically, the mounting knowledge about the many potential health benefits of vitamin D, beyond its actions on the skeleton, is occurring at the same time that vitamin D deficiency is becoming more widespread [23,24].
From the skin, cholecalciferol (vitamin D3) is transported bound to a specific vitamin D-binding alpha-globulin, which is synthesized in the liver. Also in the liver, vitamin D3 is hydroxylated, its first metabolic activation step to 25-hydroxycholecalciferol (25-OH vitamin D), which is the dominant form of both the circulating and stored vitamin D. The serum concentration of 25-OH vitamin D is an index of vitamin D nutritional status. However, vitamin D in this form is not metabolically active and for that purpose it has to be converted in the kidneys to the biologically active metabolite. The same alpha-globulin carrier protein transports 25-OH vitamin D to the kidney, the only known site of the 1-alpha-hydroxylase enzyme in the nonpregnant state, although there are extrarenal sites in pregnancy, the placenta in particular [25–27]. Hydroxylation takes place in cells of the proximal convoluted tubule producing 1α,25-(OH)2D3 which is the most potent vitamin D metabolite. Its serum concentration is 1000 times less than that of 25-OH vitamin D but, in its absence, the syndrome of vitamin D deficiency develops. As it is synthesized in the kidney, secreted into the bloodstream, and exerts its chief effects elsewhere in the body, it could correctly be regarded as a hormone.
The prime function of 1α,25-(OH)2D3 is to sustain plasma levels of calcium and phosphate which allow mineralization of newly forming bone. Vitamin D acts on at least three target organs: intestine, bone and kidney. Its major function is in the small intestine, where it induces synthesis of brush border calbindin-9K proteins that bind calcium and phosphate, promoting their active transport from the intestinal lumen to the bloodstream. In the absence of vitamin D, the intestinal absorption of these ions decreases almost completely. There is growing evidence to suggest that besides promoting intestinal absorption, skeletal mobilization, and renal tubular reabsorption of calcium, 1α,25-(OH)2D3 has many other actions. These include an antiproliferative effect on several cell types, such as keratinocytes and certain cancer cells [23,24,28]. An important effect relevant to pregnancy is related to mediation in the immune tolerance needed to maintain gestation [29,30].
The gestational increase in 1α,25-(OH)2D3 indicates that maternal 1-alpha-hydroxylase enzymatic activity is stimulated. This increase seems to be largely unrelated to parathyroid hormone (PTH) levels which decrease in early pregnancy and increase, but only to the mid-normal range, by term [31–33]. Under these conditions a lower concentration of 1α,25-(OH)2D3 should be expected. Therefore, other factors must be operating during pregnancy responsible for the upregulation of 1-alpha-hydroxylase activity and the gradual increase of 1α,25-(OH)2D3 that reaches levels that are twice as high as in nonpregnant women. These other factors include estradiol, PRL, HPL and particularly PTHrP [14,15,17]. The maternal kidneys are believed to account for most of the increased levels of 1α,25-(OH)2D3 which persists until delivery. The decidua, placenta and fetal kidneys might contribute small amounts.
Parathyroid hormone
The physiologic function of PTH is to regulate and maintain calcium concentration within narrow limits. Its effects are mediated by intracellular cyclic adenosine monophosphate (cAMP). Any fall in ionized calcium is countered by secretion of PTH. The fall in calcium is checked by the direct effects of PTH on bone and kidney, and its indirect stimulation of intestinal calcium absorption mediated by 1α,25-(OH)2D3 [34].
In bone, PTH stimulates the activity of osteoclasts and osteoblasts with a predominant effect on bone resorption and calcium and phosphate mobilization. As it stimulates secretion of osteoclast collagenases and similar lytic enzymes, it acts to accomplish metabolic destruction of the bony matrix. This is reflected in increased urinary and plasma hydroxyproline. Remodeling activity is also stimulated, but osteoclast function predominates [35].
In the kidney, PTH inhibits phosphate reabsorption by the proximal tubule. Phosphate released from bone is thereby rapidly excreted with resulting hypophosphatemia and hyperphosphaturia in states of PTH excess. PTH is also the main factor regulating 1-alpha-hydroxylase activity, the enzyme involved in the conversion of 25-(OH) vitamin D to 1α,25-(OH)2D3 which also takes place in the proximal renal tubules, although during pregnancy this activity might be predominantly exerted by PTHrP rather than by PTH. Therefore, PTH has a major effect on the renal clearance and reabsorption of calcium. If the flood of calcium from bone exceeds the capacity for calcium reabsorption, hypercalciuria ensues. PTH also inhibits proximal tubular reabsorption of bicarbonate, so that states of PTH excess are often accompanied by a mild metabolic acidosis [34].
Initial studies on PTH levels in pregnancy reported contradictory results, with some showing an increase [36,37], no change [9,38] or a decrease [39,40,41]. A previous notion that pregnancy was a state of “mild physiologic hyperparathyroidism” seems to have been an artifact of earlier, less valid measurement methods. Studies measuring the intact PTH molecule by means of a two-site immunoradiometric assay (IRMA) show a decrease to the low-normal range in the first trimester and a gradual increase to the mid-normal range by term [1,4,5,17,18,31–33,42,43].
Parathyroid hormone-related protein
This is a peptide with PTH-like activity first recognized as a protein from some malignant tumors with humoral hypercalcemia. Although different from PTH, it shares enough amino acid sequences in its structure to bind effectively to PTH receptors and to have some of its physiologic actions as well, including the bone-resorbing, phosphaturic and hypocalciuric effects [44,45].
PTHrP levels increase during the late pregnancy stages. The origin of this peptide in pregnancy is probably multifactorial and the proportional amounts contributed by each possible source have not been determined. It is produced by tissues in both the mother and fetus, including the placenta, amnion, decidua, umbilical cord, breasts and fetal parathyroid glands. It may also be involved in embryogenesis, fetal differentiation and growth [46,47], onset of labor [48], milk production [49], maternal–fetal calcium transfer [13,14,50] and protection of the maternal skeleton during pregnancy [51,52]. PTHrP is thought to contribute to the higher 1α,25-(OH)2D3 and to the lower PTH levels during pregnancy. The protective effect on the maternal skeleton is thought to be related to a five amino acid fragment in its carboxyl-terminal section [51,52]. Adequate levels of PTHrP might also be protective against premature labor because of its smooth muscle relaxant properties which favor uterine stretching. PTHrP levels fall very rapidly after the rupture of the fetal membranes, thus allowing labor to proceed [48,53].
Calcitonin
Calcitonin is secreted by the parafollicular C cells of the thyroid. Its output is stimulated by a rise in ionized calcium concentration; hypocalcemia, on the other hand, has the opposite effect. The calcium-lowering effect of calcitonin is accompanied by a fall in serum phosphate, and both probably result from a direct inhibition of bone breakdown. Calcitonin also inhibits the bone reabsorption produced by a number of substances, including PTH and vitamin D metabolites. However, in medullary carcinoma of the thyroid, where there is hypersecretion of calcitonin, there are no overt bony changes and no disturbances of calcium or phosphate levels. An intriguing observation has been that of osteopetrosis in the newborn babies of women with medullary thyroid carcinoma, but this may represent genetic linkage of two disorders rather than a calcitonin effect [54]. Calcitonin levels increase during pregnancy and it is possible that, in addition to the amounts secreted by the parafollicular C cells of the thyroid, the placenta and breast tissues may contribute to its circulating levels. The action of CT is antagonistic to that of PTH, but it is not yet known if it might act as a protector of the maternal skeleton during pregnancy. In a mouse animal model lacking CT, increased skeletal mineral content during pregnancy was not impaired [55].
Bone changes during pregnancy
Animal experiments in rodents suggest that there is a net increase of 5–10% in mineral skeletal content during pregnancy. However, studies in humans have not been clear-cut in this regard. Publications reporting studies on the serum indices of bone formation (bone-specific alkaline phosphatase, osteocalcin, procollagen I and carboxypeptidase) and urine markers of bone resorption (24-hour collection of deoxypyridinoline, hydroxyproline and pyridinoline) indicate that bone formation indices are decreased and bone resorption markers are increased. Based on those results, it has been assumed that there is decreased bone formation and accelerated bone resorption. However, these studies have been criticized because of their failure to account for the effects of the hemodilution as well as the increased GFR of pregnancy on the serum and urine concentration of those markers, among other confounding variables. Therefore they may not be accurate indicators of bone metabolism in pregnant women [17].
One prospective study using X-ray spectrophotometry showed a small but significant loss of mineral from trabecular but not cortical bone during pregnancy [56]. Ultrasonic bone propagation velocity was used in two studies scanning the proximal phalanges of fingers [57] and the os calcis [58,59] which showed a small but significant fall in bone mineral density (BMD) in the third trimester. It has also been noted that women with low calcium intake have significantly lower bone density in the third trimester than women with adequate calcium intake [57].
In a longitudinal study using dual energy X-ray absorptiometry (DEXA) in 10 women from preconception to post partum, BMD decreased 3.5% at the hip and 2% at the lumbar spine but forearm density did not change significantly [60]. Another study [61] also measured DEXA at various sites before, during and after pregnancy. BMD in pelvis and lumbar spine densities fell by 3.2% and 4.6% respectively. In a prospective cohort study of 49 women in Sweden, BMD of the forearm fell by about 1% between 12 weeks gestation and 5 months post partum. In a small study [62] of six women, BMD decreased about 3% in the femoral neck, radius and lumbar spine between 6 weeks before and 6 months after pregnancy when compared to 25 nonpregnant women matched for age, weight, height, calcium intake and activity level.
The studies using ultrasound or DEXA measurements suggest that there may be a small loss of bone mineral content of 1–4% in the pelvis and lumbar spine. However, these reports have not been able to elucidate whether or not there is an increase in BMD earlier in pregnancy in anticipation of the higher third-trimester demands when the calcium transfer to the fetus is most active and as suggested by animal studies. In addition, the postpartum measurements in some of those studies took place some time after delivery and might have measured the bone density losses that occur in the puerperium in lactating women rather than losses that took place exclusively during pregnancy.
Prospective studies using either single or dual-photon absorptiometry have not found significant change in bone density during pregnancy [5].
Changes during lactation
Human milk contains two to three times the maternal serum level of calcium, and it is estimated that between 280 and 400 mg of calcium go into breast milk daily [63]. Women breastfeeding twins might lose up to 1000 mg per day. During the first 6 months after delivery the mean calcium concentration in breast milk is slightly higher than during the succeeding 6 months but calcium loss in breast milk remains significant throughout lactation [64]. Calcium losses during lactation are even greater than in late pregnancy when calcium transfer to the fetus is greatly increased. Studies of skeletal calcium metabolism indicate that there are great demands on the maternal skeleton during lactation and bone loss may be as high as 1–3% per month [65,66].
Calcium and phosphorus
Serum levels of ionized calcium are higher than during pregnancy but do not exceed the upper normal range. Urinary calcium excretion decreases during lactation [67]. Serum phosphorus levels are also higher during lactation due to increased renal tubular reabsorption and increased bone resorption, besides the amounts obtained from the diet.
Vitamin D
The high levels of 1α,25-(OH)2D3 decrease rapidly after delivery and remain in the normal range afterwards [68].
Parathyroid hormone
Serum PTH levels are also lower during lactation but increase after weaning, sometimes even above normal. PTH is believed to play an important role in the rapid recovery of bone mass that takes place after weaning and also in the renal conservation of calcium and phosphorus [67,69].
Parathyroid hormone-related protein
The most striking of the hormonal changes observed during lactation is the marked increase in PTHrP levels in breast milk. PTHrP is produced by mammary tissue and appears to regulate calcium transfer into breast milk [70,71]. Prolactin seems to be an important factor regulating mammary PTHrP production in conjunction with local mammary factors [72]. The combination of high PTHrP and prolactin with rapidly decreasing estrogen levels is believed to be the main reason for the skeletal changes in lactating women [73]. It is estimated that lactation causes a 3–10% decline in bone mineral content after breastfeeding for 6 months, as reported by studies measuring serial bone density changes. The loss is greater in trabecular than in cortical bone [7,39,65,66]. Taller women have been reported to have greater bone loss during lactation [7,74]. Additional calcium intake does not seem to prevent bone loss during lactation or accelerate the recovery after weaning. Even in cases of low calcium intake, skeletal recovery after weaning still occurs, although there is concern that in adolescents with habitually low calcium intake, bone recovery may not be sufficient to attain peak bone mass at maturity [75].
In general, bone density is regained rapidly after weaning, and recovery of bone is complete for most women even when there are short intervals in between pregnancies [76,77].
Calcitonin
Calcitonin seems to have an important role during lactation acting as protector of the maternal skeleton against excessive bone resorption during this period of rapid demineralization [78].
Diagnosis
Vitamin D deficiency in the general population appears to be more widespread than previously thought. Insufficient amounts of vitamin D alter calcium and phosphorus metabolism and cause diminished mineralization of the collagen matrix, which results in rickets in children and osteomalacia in adults [23]. The many extraskeletal actions of vitamin D have already been described above.
Vitamin D deficiency is generally defined as a 25-hydroxyvitamin D serum level of <20 ng/mL (<50 nmol/L). Levels of 21–29 ng/mL (50–75 nmol/L) point to relative insufficiency and ≥30 ng/mL (>75 nmol/L) are indicative of sufficient amounts. Levels of 150 ng/mL (375 nmol/L) or higher are considered toxic [79,80].
Deficiency of vitamin D has also been reported to occur frequently during pregnancy and lactation [29,81–83]. Vitamin D deficiency in utero may cause growth retardation, skeletal deformities, and impaired first-year growth as well as teeth enamel defects and higher risk of fractures later in life. Neonatal hypocalcemia and, rarely, even convulsions from tetany have also been reported [84–86]. Overt clinical osteomalacia with bone fractures in the mother, or delivery of an infant with florid rickets at birth, has been described as presenting features of vitamin D deficiency [87–91], but these are now unusual presentations. More often, the vitamin D deficiency is less severe and usually there are no overt maternal clinical symptoms. However, even in these milder cases the fetus develops in a state of hypovitaminosis D which is likely to have an effect on immune function, fetal and childhood bone development and increased risk of other childhood chronic conditions [23].
Vitamin D insufficiency appears to be common even in otherwise healthy appearing pregnant women [29,81–83,92]. Some women are more likely to have children with complications, and it has been reported that 50% of the newborns of strict vegetarians have hypocalcemia [84,93–95]. Other causes of vitamin D deficiency include reduced skin synthesis because of lack of exposure to sunlight when residing in certain geographical latitudes, from sunscreen use or from wearing clothing that completely covers the skin [96].
Other causes of vitamin D deficiency include the following.
Decreased bio-availability
Decreased bio-availability of vitamin D may occur because of intestinal malabsorption in cases of celiac disease, cystic fibrosis, Whipple’s disease, Crohn’s disease, intestinal bypass surgery and medications that reduce cholesterol absorption [97]. In obesity vitamin D may remain sequestered in body fat. Obese pregnant women and their neonates have been reported to have increased risk of vitamin D deficiency [98].
Increased catabolism
Long-term use of certain medications including anticonvulsants (e.g. phenytoin, carbamazepine, phenobarbital), glucocorticoids, some anti-AIDS medications, antirejection medications and the antimicrobial agent rifampin can cause vitamin D deficiency by inducing the catabolism of 1α,25-(OH)2D3. They do so by activating the steroid and xenobiotic (foreign substances found in the body) receptor known as pregnane X receptor. Pregnane X receptor is a nuclear receptor that senses and responds to the presence of foreign toxic substances by upregulating the expression of proteins involved in their detoxification and clearance [99–104]. This effect can be significant enough to cause osteomalacia.
Decreased synthesis
Vitamin D deficiency may occur in cases of advanced liver disease when there is significant hepatic dysfunction. In these cases there is decreased synthesis of 25-hydroxyvitamin D. With less severe liver dysfunction, vitamin D production is still possible but intestinal malabsorption will occur [96].
In chronic kidney disease there is decreased synthesis of 1α,25-(OH)2D3 which may occur with creatinine clearance rates as high as 31–89 mL/min (0.5–1.49 mL/s) but particularly in more severe cases when the GFR decreases to <30 mL/min (<0.5 mL/s). Under these conditions there is hypocalcemia, secondary hypoparathyroidism, and renal bone disease [105]. In hyperphosphatemia there is decreased activity of the enzyme 25-hydroxyvitamin D-1-alpha-hydroxylase caused by increased fibroblast growth factor 23, resulting in lower serum levels of 1α,25-(OH)2D3 [106].
Increased urinary loss
Vitamin D deficiency may be seen in cases of nephrotic syndrome with heavy proteinuria when 25-hydroxyvitamin D3 bound to its alpha-globulin carrier protein is lost in the urine [107].
Hereditary disorders
The various forms of hereditary rickets may affect 1α,25-(OH)2D3 either by decreasing its synthesis (pseudovitamin D deficiency rickets or vitamin D-dependent rickets type 1) or by causing partial or complete resistance to its action (vitamin D-resistance rickets or vitamin D-dependent rickets type 2 and vitamin D-dependent rickets type 3). In autosomal dominant hypophosphatemic rickets and X-linked hypophosphatemic rickets, there is decreased 1-alpha-hydroxylase activity resulting in low levels of 1α,25-(OH)2D3 [108,109].
Acquired disorders
Acquired causes of osteomalacia include tumors secreting fibroblast growth factor 23 which results in phosphaturia, decreased intestinal absorption of phosphorus, hypophosphatemia and decreased activity of the enzyme 25-dihydroxyvitamin D-1-alpha-hydroxylase. Some lymphomas cause increased macrophage conversion of 25-OHD to 1α,25-(OH)2D3 which is the same mechanism by which granulomatous disorders cause disruption of calcium metabolism. Under these conditions the 25-OHD levels are lower and the 1α, 25-(OH)2D3 levels are increased. In hyperparathyroidism the excess PTH levels also cause increased metabolism of 25-OHD to 1α,25-(OH)2D3 and as a consequence the serum levels of the former decrease and those of the latter will be in the high normal range or elevated. Hyperthyroidism also enhances 25-OHD metabolism, which will reduce its serum levels [106–108,110].
Lactation
Infants who are exclusively breastfed and have no other vitamin D source will develop vitamin D deficiency if there are insufficient amounts in breast milk [79,108,111].
Prevention of vitamin D deficiency
Many recent studies have reported that vitamin D deficiency has become widespread and emphasize that the replacement doses should be higher than those that have been previously recommended [23,24]. However, the optimal vitamin D dosages required to achieve meaningful clinical outcomes are still under investigation. Until more specific and widely accepted recommendations are issued, this topic should be considered to be in a state of transition.
Breastfed infants and children
Human milk does not contain much vitamin D (20 IU/L) in mothers who are vitamin sufficient, and even less in women who are vitamin D deficient. Administering relatively high doses to the mother (e.g. 4000 IU/day) has been reported to allow the transfer of enough vitamin D via breast milk to satisfy the infant’s needs [111]. However, administering the vitamin D directly to the infant, rather than relying only on the vitamin D content in breast milk, is considered more effective because of the limited transfer of vitamin D through breast milk. In Canada, current guidelines recommend that all infants and children be given 400 IU of vitamin D3 daily [112]. In the USA, the American Academy of Pediatrics recommends 200 IU of vitamin D3 daily for breastfed infants [113]. However, it has been pointed out that 200 IU/day may not be enough [114] given the accumulating evidence that insufficient vitamin D in childhood is associated with an increased risk of several chronic conditions [23,24].
Pregnant or lactating women
The only vitamin D supplement taken by the majority of US women is the 400 IU contained in the prenatal vitamin preparations. However, vitamin D deficiency is still common by the end of pregnancy even in women who take prenatal vitamins [83]. It is generally agreed that the current recommendations (200–600 IU/day) [115] are not enough and that the requirements may be 1000 IU/day [79] or more [81]. Higher dosages, 4000 IU/day or 60,000 IU/month of vitamin D2, were more effective than lower doses in preventing hypovitaminosis D in lactating women and without reports of toxicity [81,82,111,116].
Reduced skin synthesis
The 1000–2000 IU of vitamin D3/day recommended for pregnant and lactating women might also be sufficient for those lacking exposure to adequate amounts of sunlight [96,117] although doses >2000 IU/day may be more desirable and have been reported to be safe and effective in men and nonpregnant women [118,119].
Decreased bio-availability from malabsorption syndromes
In addition to sensible sunlight or ultraviolet B radiation exposure, the doses recommended for these conditions are up to 10,000 IU of vitamin D3/day. These dosages have been reported to be safe, effective and without evidence of toxicity when taken for as long as 5 months [117,120–122]. An alternative dosing could be 50,000 IU of vitamin D2/week. In obesity, 1000–2000 IU of vitamin D3/day or 50,000 IU of vitamin D2 every 1–2 weeks are the recommended preventive doses to avoid deficiency [96].
Increased catabolism
Patients taking anticonvulsants and other medications that activate the steroid and xenobiotic receptor [98–103], as well as antirejection medications used after transplantation, are recommended to take 50,000 of vitamin D2 every other day or every week.
Liver failure
The dosages recommended for situations of reduced synthesis should be followed for these patients as well. If malabsorption is also present, the recommendations given above should be advised [96,107].
Increased urinary loss: nephrotic syndrome
The recommended doses are 1000–2000 IU/day of vitamin D3 or 50,000 IU of vitamin D2 every 2–4 weeks [96,105,107,123,124].
Chronic kidney disease
Control of serum phosphate is necessary [107]. Vitamin dosing is 1000 IU/day of vitamin D3 or 50,000 IU of vitamin D2 every 2 weeks [105,123]. Once vitamin D sufficiency is obtained (30–60 ng/mL), a vitamin D analog may be needed in the milder renal insufficiency cases (creatinine clearance 31–89 mL/min or 0.4–1.49 mL/s) and generally recommended when the GFR is 30 mL/min (0.5 mL/s) or less. Calcitriol 0.25–1.0 μg once or twice a day is more often used, but other active vitamin D analogs may be utilized as well [123,124].
Hereditary disorders
In X-linked hypophosphatemic rickets and autosomal dominant hypophosphatemic rickets, in order to improve bone mineralization, phosphorus levels need to be brought up to normal. Calcitriol (0.5–1 μg/day) and oral phosphate (1–4 g/day in divided doses) are effective, but close monitoring is needed to avoid renal insufficiency and nephrocalcinosis. Vitamin D-dependent rickets type 1 responds to calcitriol 0.5–1 μg/day, and calcium and phosphorus supplements are generally not needed. In vitamin D-dependent rickets type 2 and 3, there is end-organ resistance to 1α,25-(OH)2D3. These patients require pharmacologic doses of calcitriol (5–30 μg/day) as well as mineral supplementation. Even so, some patients may have little or no response even to these high calcitriol doses [108,109].
Acquired disorders
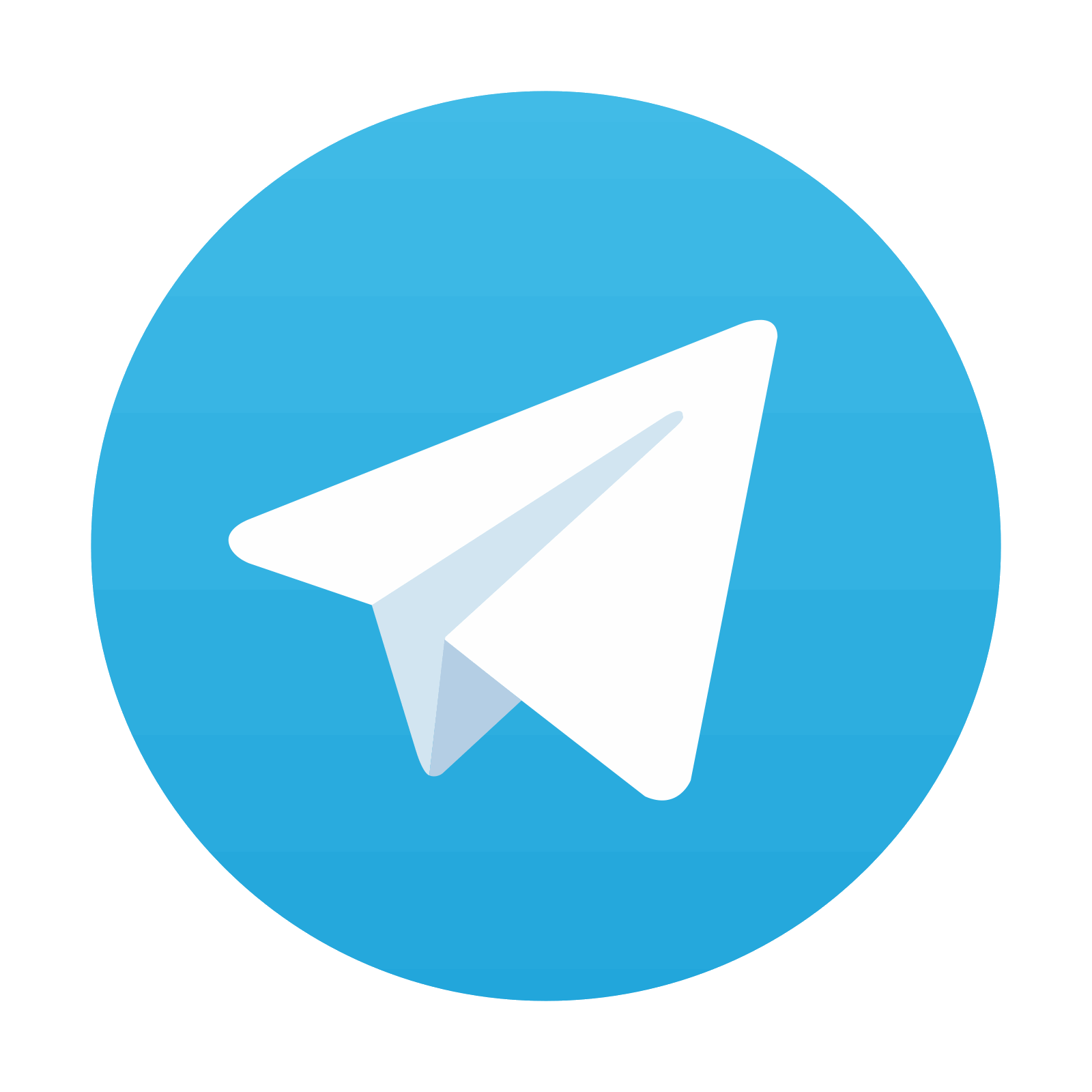
Stay updated, free articles. Join our Telegram channel
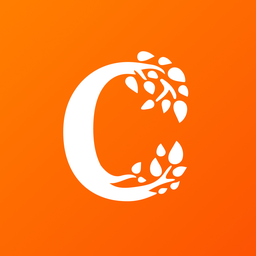
Full access? Get Clinical Tree
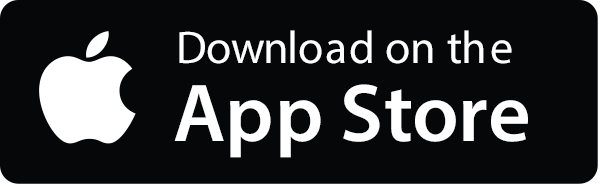
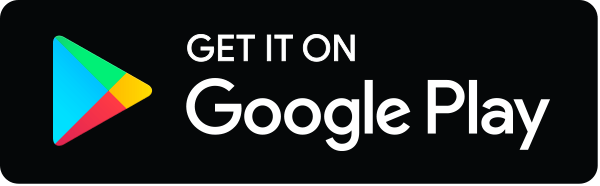