Respiratory medicine
Will Carroll, Warren Lenney
• Know about the embryology of the respiratory system
• Know the anatomy of the respiratory system
• Understand the physiology of respiration
• Know the physical signs and symptoms and presentation of respiratory illness
• Understand the changes in the respiratory system during sleep
• Be able to select and interpret appropriate respiratory investigations
• Know the aetiology and management of respiratory diseases of childhood
• Understand the pharmacology of agents commonly used in those diseases
• Know the respiratory complications of other system disorders
Embryology
The respiratory tree arises from the ventral surface of the foregut. The phases of lung development are shown in Figure 17.1. Abnormal embryological development may result in congenital thoracic malformations (CTMs), which include:
• Congenital pulmonary airway malformations (CPAM), previously known as congenital cystic adenomatoid malformations (CCAM), from abnormal development of alveolar or bronchial tissues
• Pulmonary sequestration from an abnormal blood supply to part of the lung (usually systemic rather than pulmonary)
• Congenital diaphragmatic hernia from maldevelopment of the pleuroperitoneal canal with or without associated deficiency of the diaphragm itself
• Cysts, bronchogenic or foregut
• Congenital lobar emphysema from partial obstruction of the developing airway, most commonly due to a deficiency of bronchial cartilage development
• Lung agenesis/bronchial atresia from maldevelopment of the tracheobronchial tree in early fetal life.
Anatomy
The respiratory system is split into three major parts: the upper airways, the conducting airways and the lower respiratory tract. Whilst they are smaller than the final adult configuration and the relative size of the component parts is somewhat different (e.g. the epiglottis is relatively large and floppy) at birth, the upper and conducting airways are fairly well developed. In contrast, the lower respiratory tract is significantly different from the adult constitution with far fewer alveoli and proportionately more conducting airways.
The upper airway (Fig. 17.2) is made up of the nasal cavity (the nose is the natural airway for all of us but young children in particular require a clear nasal passage to breathe), sinuses, the pharynx and the larynx. The entire upper airway and the conducting airways are lined with ciliated (respiratory) epithelium. Abnormalities in the cilia lead to blockage and obstruction, as these are required to ensure clearance of mucus (see Ciliary dyskinesias, below).
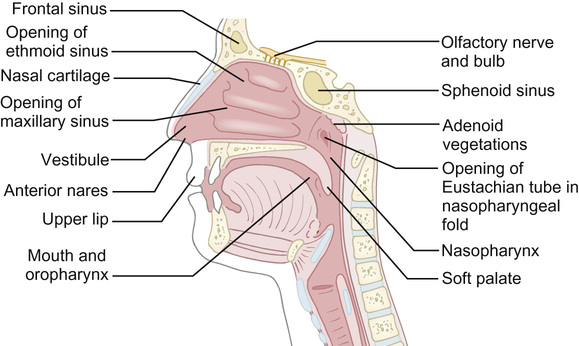
The main functions of the nasal portion of the airway are filtration, humidification and warming. Particles larger than 10 µm in diameter tend to impact in the upper airway and most are not inhaled into the lower airway. The air reaching the trachea varies in temperature from around 6 to 30°C depending upon the minute ventilation, size of the child and ambient temperature. It is warmed to 37°C in the trachea and lungs. Heat from gases expired through the nose is extracted by use of a countercurrent exchange system.
Loss of control of the pharyngeal muscles, either due to pathology (e.g. cerebral palsy, muscular dystrophies) or deep sedation, leads to intermittent upper airway obstruction (stertor). Narrowing at the larynx leads to fixed upper airway obstruction (stridor – see later). In babies, the airway is maximally open when the head is held in a neutral position. As the relative size of the head decreases, the airway becomes maximally opened with increasing degrees of neck extension.
The conducting airways are made up of the trachea, bronchi and bronchioles that make up the first sixteen branches of the tracheobronchial tree. Cartilage is an important constituent of the larynx and the conducting airways and at birth this is significantly more floppy than in later life.
The trachea divides into two bronchi. The right bronchus is wider than the left and it is more vertical. Therefore, inhaled objects tend to fall into the right main bronchus. The bronchi divide into four lobar bronchi, which, in turn, subdivide into 16 segmental bronchi. After 16 subdivisions, we reach the terminal bronchioles which are capable of limited gas exchange.
The lower airways (divisions 16–23 of the airways) are where most gas exchange takes place. The formation of the lower airways is only partly complete at birth and alveolarization (the formation of new alveoli) continues until at least 2 years of age.
The lungs are surrounded by the visceral pleura. This is separated by a thin layer of pleural fluid from the parietal pleura, which covers the thoracic walls and upper surface of the diaphragm. Due to the considerable surface tension that exists, the pleura usually remain closely opposed. However, they can still slide easily over each other (think of two glass microscope slides separated by a little fluid – they slide easily over each other but are hard to pull apart). Fusion of the two layers by the production of fibrin leads to pain on movement (pleurisy). Separation of the pleura by either fluid (pleural effusion) or gas (pneumothorax) can also lead to pain, particularly on inspiration.
Physiology
Airway resistance and compliance
Airway resistance is a result of the frictional force which opposes the flow of air. Airway resistance must be overcome for air to flow. The resistance for laminar flow (most airflow in the airways is laminar under normal conditions) is described by Poiseuille’s equation:

This predicts that resistance increases dramatically as diameter decreases. However, most resistance to airflow is offered by the trachea and larger bronchi. Although counterintuitive, this is due to the branching of the tracheobronchial tree, which means that the combined cross-sectional area of the smallest airways is sufficiently large to provide little resistance to flow. Whilst the airway diameter at the 23rd branching is only 0.4 mm in diameter, the total cross-sectional area is 4 m2 as there are 300 million airways of this size. In contrast, the tracheal cross-sectional area is just 3 cm2. Thus, under normal circumstances, most of the total airway resistance is offered by just 10% of the total lungs – the large conducting airways. This becomes important when considering the results of lung function testing (spirometry) – see below.
In young children, the decreased size of the airways results in overall increased resistance to flow. This is coupled with a reduction in chest wall compliance. Under these circumstances the chest wall can become drawn inwards with each breath even in health. Obviously, this worsens if there are any additional factors that further increase airways resistance (e.g. bronchiolitis).
The compliance of the lung is a measure of how easily it can be distended. It is given by the formula:

During the first part of inspiration, a relatively greater pressure is required to generate airflow. Therefore, compliance varies depending upon the exact lung volume.
Compliance also varies with age. A newborn child has stiff lungs with very low compliance compared to a young adult. A typical adult male will have lung compliance of between 0.09 and 0.26 L/cmH2O, whilst a newborn infant will have lungs that are more than 20 times less compliant with values of 0.005 L/cmH2O.
Control of breathing
The primary control of breathing (Fig. 17.3) is via the autonomic nervous system. It is mediated mainly, but not exclusively, through neural centres in the brainstem. Recent studies have shown, however, the existence of other chemosensitive cells in, for example, the hypothalamus and the cerebellum, that are exquisitely sensitive to changes in hydrogen ion concentration. Carbon dioxide in the blood diffuses rapidly into the cerebrospinal fluid, where it reacts with water to release hydrogen ions. This accounts for the rapid respiratory response to changes in arterial carbon dioxide levels. Afferent input also occurs via peripheral chemoreceptors sensitive both to oxygen and carbon dioxide, which are located in the carotid and aortic bodies, irritant receptors in the upper and lower airways and mechanical receptors in the lungs and chest wall. These receptors facilitate the response to hypoxia, which is much less rapid than the response to carbon dioxide. As excess H+ will only cross the blood–brain barrier slowly, therefore metabolic acidosis tends to be incompletely and slowly corrected. Autonomic control can be overridden by conscious control enabling, for example, speech and breath-holding to occur. Voluntary conscious signals are generated in the cortex and conducted to muscles of breathing via the corticospinal tract.
The rate and depth of normal quiet (tidal) breathing are controlled within the brainstem. During tidal breathing, the distribution of air within the lungs is determined by regional variation in airway resistance and lung compliance. In an upright child, the weight of the lungs ensures that the pleural pressure is more negative at the apex. This leads to relative over-distension at the apices with relatively reduced volumes at the bases. This is most prominent in infancy and early childhood but is opposed by the rigid chest wall and stronger respiratory muscles in adult life. During infancy, any increases in airway resistance (usually as a result of airway narrowing, e.g. smooth muscle thickening and/or airway cell oedema) will result in a greater effort of breathing. The relatively weak intercostal muscles and compliant chest wall are unable to oppose the stronger contraction of the diaphragm, leading to the abdominal breathing and sternal/intercostal recession seen in infants with respiratory distress.
The role of the diaphragm
The major muscle of respiration is the diaphragm. At birth, fatigue-resistant striated muscle fibres account for only 10% of its muscle mass. This increases to 50% by early adulthood. Infants, therefore, will tire more quickly and are at increased risk of apnoea and respiratory failure if the work of breathing needs to increase for more than a few minutes for any reason e.g. bronchiolitis or heart failure due to ventricular septal defect (VSD). The more compliant chest wall predisposes the immature airway to partial closure, particularly at the lung bases, leading to intrapulmonary shunting of blood through non-ventilated areas.
The diaphragm and accessory muscles are affected differently by sleep. During sleep, diaphragmatic function is largely preserved. This is essential for maintenance of adequate ventilation. Accessory muscle function is reduced, particularly during REM sleep. This may contribute to hypoventilation and ventilation–perfusion mismatching resulting in oxygen desaturation.
Positioning and oxygenation
The matching of ventilation with perfusion takes place within the airways and alveoli. Sudden changes cannot be instantly compensated for and may lead to ventilation–perfusion mismatch. The clinical consequences are not always obvious. Placing a child with respiratory distress in a horizontal position (lying them flat) leads to an immediate reduction of ventilation in the dependent lungs. This is not immediately accompanied by a change in lung perfusion, causing mismatch and potentially decreasing oxygen saturation levels.
Minute volume, tidal volume and lung capacities
In normal breathing, the volume of inspired air and expired gases that move in and out of the lungs each minute (minute volume) is a product of the volume of each breath (tidal volume) and the respiratory rate (breaths per minute).
From the age of five, lung volume changes can be measured using a spirometer (Fig. 17.4). The vital capacity (VC) and the forced expiratory volume in 1 second (FEV1) can be measured spirometrically, but total lung capacity (TLC), functional residual capacity (FRC) and residual volume (RV) require different techniques (see below).
Gas exchange and diffusion
Exchange of gas across a surface is dependent upon the gradient of partial pressure across it, the surface area, and the magnitude of the diffusion distance. Gas transfer occurs by diffusion. Carbon dioxide is very water-soluble so diffuses more readily than oxygen across the membranes and is less affected by increases in diffusion distance, as occurs in pulmonary oedema. The differential changes seen in blood carbon dioxide and oxygen content in respiratory failure allow us to understand where a problem is likely to be occurring (Table 17.1). Children may present with a combination of problems.
Table 17.1
Causes of hypoxia and their response to oxygen supplementation
Cause of hypoxia | Effect of increase in FiO2 | Arterial CO2 |
Alveolar hypoventilation | Correction of hypoxaemia | Increased |
Impaired diffusion | Correction of hypoxaemia | Normal or decreased |
Right–left shunt or ventilation–perfusion imbalance | Little change or no change | Decreased |
Oxygenation of blood
Whilst some oxygen is dissolved in the plasma (3 ml/L of arterial blood) the vast majority is transported bound to haemoglobin. Oxygen saturation describes the percentage of haemoglobin molecules that are bound to oxygen. When all the possible sites for oxygen binding within haemoglobin are occupied, oxygen saturation is 100%. Oxygen saturation and oxygen content of blood do not share a linear relationship. The relationship is shown by the oxygen dissociation curve (Fig. 17.5). The sigmoidal shape demonstrates how haemoglobin is an effective carrier of oxygen.
Physiology of respiratory signs
The rate and pattern of breathing
Normal tidal volume is proportional to weight throughout life; usually 6–8 mL/kg. Energy requirements and therefore oxygen demand and carbon dioxide production are relatively higher in younger children. Respiratory rate is therefore higher in younger children and decreases with age.
The normal breathing pattern in children is variable. In an awake child, it is modulated by various baseline physical activities and is under conscious control. During sleep, physiological changes occur that vary with sleep state and are influenced by maturational changes in respiratory mechanics and control of breathing. Studies of healthy infants show that pauses and gaps in breathing up to 15 seconds during sleep are common but may be exaggerated in illness. The response to hypoxia is biphasic in infants, with an initial increase in respiratory rate followed by a decrease, often in association with apnoea.
In well children, minute ventilation falls 15% during sleep. In addition, during rapid eye movement (REM) sleep, there is an automatic decrease in accessory muscle activity accompanied by an increase in upper airway resistance as the muscles supporting the upper airway relax. Newborn infants sleep for approximately two thirds of the time, 60% spent in REM-equivalent sleep. By six months of age, most children sleep 11–14 hours per day, one third being REM sleep. The typical adult sleeps 8 hours a day, 20% being in REM sleep.
Grunting and forced expiratory braking
It would be inefficient for airways and lungs to collapse completely during expiration, as re-inflation requires much more energy than widening/expanding a partially closed airway. Under normal circumstances, airway closure is opposed by maintenance of functional residual capacity (FRC) at a level above the point at which airways collapse. Collapse is increased in surfactant deficiency, bronchiolitis and pneumonia. FRC is reduced when lying supine, during anaesthesia and sleep.
Three factors influence the FRC: elastic recoil of the lung, the time allowed for expiration and the expiratory flow rate. Elastic recoil increases with age during childhood, making older children less susceptible to complete airway closure. Younger children oppose airway collapse by having higher respiratory rates, thus reducing the time allowed for expiration. If this is insufficient to maintain FRC then infants will attempt to reduce expiratory flow rates using partial closure of the glottis or upper airway. This leads to grunting, as seen in neonates with respiratory distress, where glottic closure maintains a positive expiratory pressure but reduces expiratory flow.
Cough
Reflex or voluntary coughing requires coordination of a complex series of events. It begins with opening the glottis and a short inspiration to increase lung volume. Recruitment of lung volume is essential to maximize expulsion, as this allows elastic recoil of the lung to assist muscular contractions. Next, the glottis closes and respiratory muscles contract, generating high intra-thoracic pressures. Shortly after closure, the glottis re-opens causing rapid decompression of the airways and a high velocity expulsion of gas. These rapid shifts in airway volume also cause a degree of small airway closure and compression, physically squeezing mucus and other intraluminal contents into the larger proximal airways. As expired air flows from the alveoli to the mouth, pressure within the airway falls. If there is airway obstruction, this pressure drop is greater, with a tendency for more proximal airways to collapse. This flow-related collapse during a forced expiration accounts for the brassy sound that occasionally occurs during maximal forced expiration in healthy subjects. It is more common if there is narrowing of the larger airways or if they are less rigid than usual as in bronchomalacia.
Cough is an important symptom in childhood. It is often informative to hear a cough (or see video recording of a cough), allowing one to distinguish between dry and wet coughs and wheezy or brassy coughs. Wet coughs suggest increased mucus within the airways, whereas the presence of wheeze suggests smaller airway obstruction. A brassy or barking cough suggests narrowing of the larger airways (this results in the barking cough of croup) and/or a degree of bronchomalacia.
Children with muscular weakness have reduced ability to cough. It is possible to measure the force of coughing by asking the child to cough as hard as they can into a peak flow meter, either using the mouthpiece or a mask. A cough expiratory flow of >270 L/minute in adults or older children predicts adequate cough strength. Physiotherapy may assist airway clearance in children with inadequate cough strength. Cough assist devices work on the same principle by generating a positive pressure followed by a negative pressure upon initiation of cough. They assist complete expansion of the airways and chest. This increases airway clearance as the natural elastic recoil rapidly empties the chest. At the same time a degree of negative pressure is applied via a tightly applied face mask. With cooperation and practice, most children with weak cough find this a useful aid.
The cough reflex itself has peripheral and central components. Cough receptors exist throughout the respiratory system. There are irritant receptors in the larynx and large airways and stretch receptors within the lung parenchyma that stimulate cough in response to mechanical irritation or over-distension of the lung. The cough pathway involves cough receptors, mediators of sensory nerves, an afferent limb, the vagus nerve, the central cough centre and an efferent limb.
Normal breath sounds, crackles, stridor and wheeze
Normal breath sounds are described as vesicular. Dampening of the sound as it travels through normal lung tissue ensures that, as the lung deflates and flow diminishes, there is little or no sound towards the end of expiration (Fig. 17.6). In pneumonia, the lung parenchyma becomes filled with inflammatory cells and fluid, which conduct sound from the central airways more efficiently. Expiration is longer and the breath sounds on auscultation harsher and similar to airflow heard by listening over the trachea.
Crackles are discontinuous, non-musical additional respiratory sounds. There are two types of crackles. Coarse crackles are the easiest to hear, occurring when fluid or secretions collect in the large airways. In addition, coarse bubbling sounds can be heard that often clear or alter after coughing or taking a deep breath. Fine crackles or crepitations are higher pitched, explosive sounds. They are thought to occur when small closed airways suddenly open during inspiration. They are often obscured by other respiratory noises and are dampened by hyperinflation or the thicker chest wall of older children.
Wheeze is primarily heard during expiration and is a musical sound caused by flow limitation in the small intra-thoracic airways. It represents oscillation of the airway walls at the site of narrowing. In severe airway obstruction, wheezing also occurs in inspiration.
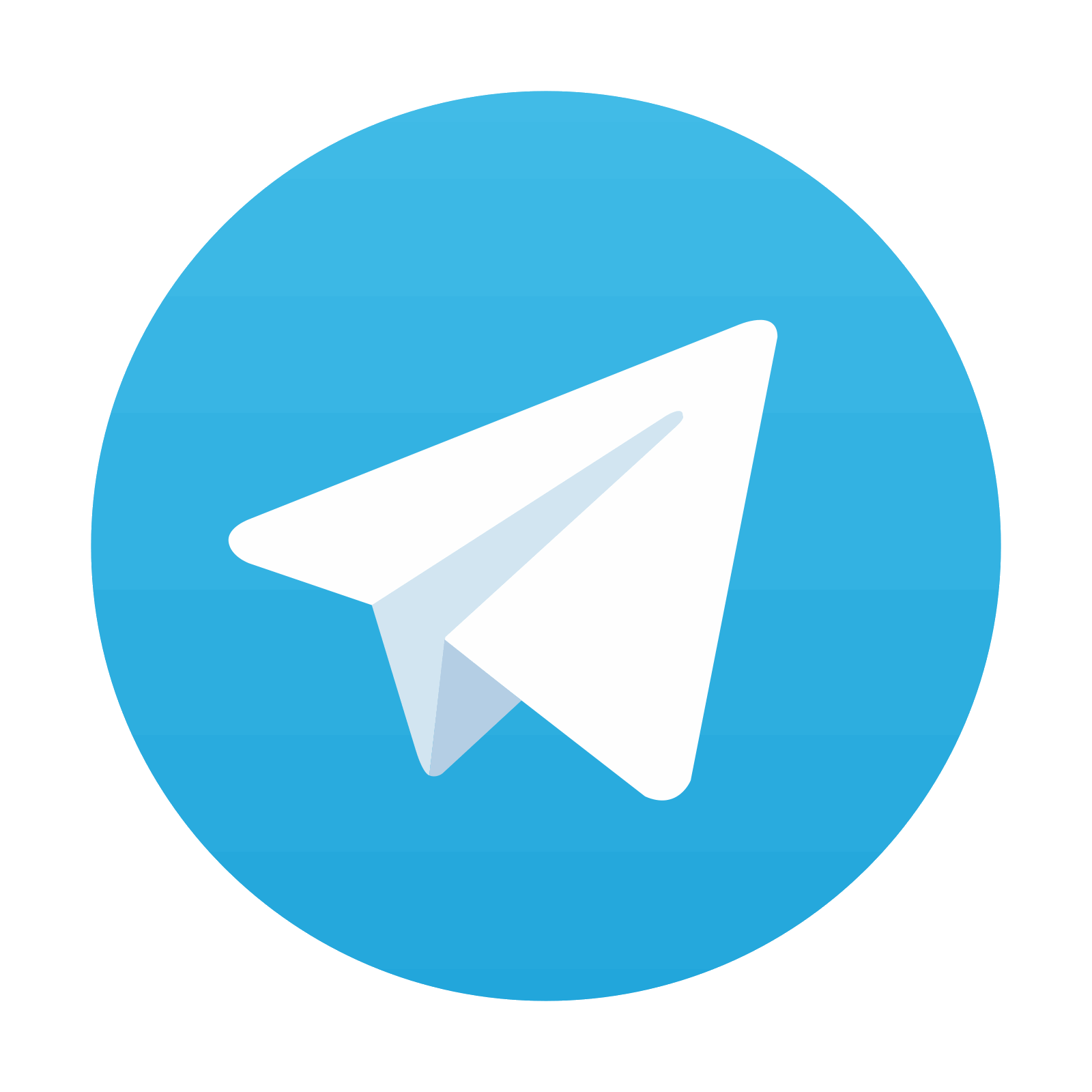
Stay updated, free articles. Join our Telegram channel
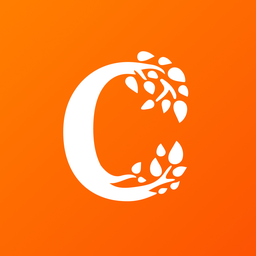
Full access? Get Clinical Tree
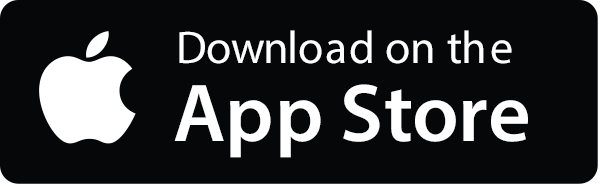
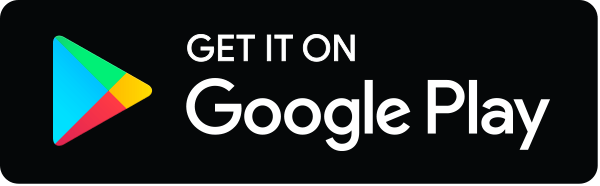