Respiratory Distress Syndrome
Kushal Y. Bhakta
The primary cause of respiratory distress syndrome (RDS), formerly known as hyaline membrane disease, is inadequate pulmonary surfactant. Preterm birth is the most common etiologic factor. The manifestations of the disease are caused by the resultant diffuse alveolar atelectasis, edema, and cell injury. Subsequently, serum proteins that inhibit surfactant function leak into the alveoli. The increased water content, immature mechanisms for clearance of lung liquid, lack of alveolar-capillary apposition, and low surface area for gas exchange typical of the immature lung also contribute to the disease. Prenatal diagnosis to identify infants at risk, prevention of the disease by antenatal administration of glucocorticoids, improvements in perinatal and neonatal care, advances in respiratory support, and surfactant replacement therapy have reduced mortality from RDS. However, RDS remains an important contributing cause of neonatal mortality and morbidity, especially among the most immature infants.
I. IDENTIFICATION
-
A. Perinatal risk factors
-
Factors that affect the state of lung development at birth include prematurity, maternal diabetes, and genetic factors (white race, history of RDS in siblings, male sex). Thoracic malformations that cause lung hypoplasia, such as diaphragmatic hernia, may also increase the risk of surfactant deficiency. Genetic disorders of surfactant production and metabolism include surfactant protein B and surfactant protein C gene mutations, and mutations of the ABCA3 gene, whose product is an adenosine triphosphate (ATP)-binding cassette transporter localized to the lamellar bodies of alveolar type II cells. These rare disorders cause a severe RDS-like picture, often in term infants, and are usually fatal without lung transplantation.
-
Factors that may acutely impair surfactant production, release, or function include perinatal asphyxia in premature infants and cesarean section without labor. Infants delivered before labor starts do not benefit from the adrenergic and steroid hormones released during labor, which increase surfactant production and release. As a result, RDS may be seen in late preterm or early term infants delivered by elective cesarean section.
-
-
Prenatal prediction
-
Assessment of fetal lung maturity (FLM). Prenatal prediction of lung maturity can be made by testing amniotic fluid obtained by amniocentesis.
-
The lecithin/sphingomyelin (L/S) ratio is performed by thin-layer chromatography. Specific techniques vary among laboratories and may affect the results. In general, the risk of RDS is very low if the L/S ratio is >2. Exceptions to the prediction of pulmonary maturity with an L/S ratio >2 are infants of diabetic mothers (IDMs), infants with erythroblastosis fetalis, and infants who have suffered intrapartum asphyxia. Possible exceptions are intrauterine growth restriction (IUGR), abruptio placentae, preeclampsia, and hydrops fetalis. Contaminants, such as blood and meconium, affect the interpretation of results.
-
The TDx-FLM II measures the surfactant to albumin ratio using fluorescent polarization technology. A value of >55 mg surfactant/g albumin correlates with lung maturity; the predictive capacity of the test may improve if gestational age—specific threshold values are used. Contamination with blood or meconium may interfere with interpretation of this test.
-
Lamellar body counts in the amniotic fluid have also been used as a rapid and inexpensive test to determine FLM. Lamellar bodies are “packages” of phospholipids produced by type II alveolar cells and are present in amniotic fluid in increasing numbers with advancing gestational age. A value of >50,000 lamellar bodies/microliter predicts lung maturity. Lamellar bodies can also be assessed indirectly by measuring optical density of amniotic fluid.
-
The presence of phosphatidylglycerol (PG) can also be used to determine FLM, but PG appears late in the maturation process of the lung. An advantage to this test is that it is not affected by contamination with blood or meconium. The major disadvantage is that its sensitivity is low, and thus can give a falsenegative result when other tests indicate lung maturity.
-
Foam stability index (FSI) predicts FLM based on the formation of a stable foam when amniotic fluid is shaken with ethanol in a test tube. Blood and meconium contamination interfere with interpretation of this test; interpretation may also vary among users.
-
-
Antenatal corticosteroid therapy should be given to pregnant women 24 to 34 weeks’ gestation with intact membranes or with preterm rupture of the membranes (ROM) without chorioamnionitis who are at high risk for preterm delivery within the next 7 days. The efficacy of treatment at gestational ages earlier than 24 weeks is uncertain; however, administration below this age may be reasonable depending upon clinical circumstances. This strategy induces surfactant production and accelerates maturation of the lungs and other fetal tissues, resulting in a substantial reduction of RDS, intraventricular hemorrhage (IVH), necrotizing enterocolitis (NEC), and perinatal mortality. A full course consists of two doses of betamethasone (12 mg IM) separated by a 24-hour interval, or four doses of dexamethasone (6 mg IM) at 12-hour intervals, although incomplete courses may improve outcome. Contraindications to treatment include chorioamnionitis or other indications for immediate delivery. Most studies suggest that betamethasone may be preferable because of potential neurotoxicity of dexamethasone. However, the only randomized trial (Betacode trial) comparing both drugs found no difference in most outcomes, except that the rate of IVH and brain lesions was higher in infants exposed to betamethasone.
-
-
Postnatal diagnosis. A premature infant with RDS has clinical signs shortly after birth. These include tachypnea, retractions, flaring of the nasal alae, grunting, and cyanosis. The classic radiographic appearance is of low-volume lungs with a diffuse reticulogranular pattern and air bronchograms.
II. MANAGEMENT.
The keys to the management of infants with RDS are (i) to prevent hypoxemia and acidosis (this allows normal tissue metabolism, optimizes surfactant production, and prevents right-to-left shunting); (ii) to optimize fluid management (avoiding hypovolemia and shock, on the one hand, and edema, particularly pulmonary edema, on the other); (iii) to reduce metabolic demands; (iv) to prevent worsening atelectasis and pulmonary edema; (v) to minimize oxidant lung injury; and (vi) to minimize lung injury caused by mechanical ventilation.
-
Oxygen
-
Delivery of oxygen should be sufficient to meet target saturation values, although the range of optimal oxygenation is uncertain. For infants who require supplemental oxygen, one approach is to target SpO2 in the 88% to 92% range for infants less than 30 weeks’ gestation or 1,250 g (set monitor alarm limits 85%—95%). For infants 30 weeks’ gestation or more, or when infants reach 30 weeks’ postmenstrual age, target SpO2 from 88% to 95% (set alarm limits 85%—97%). If these targets are maintained, arterial PO2 rarely will exceed 90 mm Hg. (see Chap. 30, Section II.D.1.c. Targeted saturation values). Higher than necessary fractional concentration of inspired oxygen (FiO2) levels should be avoided because of the danger of potentiating the development of lung injury and retinopathy of prematurity. The oxygen is warmed, humidified, and delivered through an air-oxygen blender that allows precise control over the oxygen concentration. For infants with acute RDS, oxygen is ordered by concentration to be delivered to the infant’s airway, not by flow, and oxygen concentration is checked at least hourly. It should be titrated to the targeted oxygen saturation, which should be monitored continuously. When hand ventilation is required during suctioning of the airway, during insertion of an endotracheal tube, or during an apneic spell, the oxygen concentration should be similar to that before bagging to avoid hyperoxia and should be adjusted in response to continuous monitoring.
-
Blood gas monitoring (see Chap. 30). During the acute stages of illness, frequent sampling may be required to maintain arterial blood gases within appropriate ranges. Arterial blood gases (arterial oxygen tension [PaO2], arterial carbon dioxide tension [PaCO2], and pH) should be measured 30 minutes after changes in respiratory therapy, such as alteration in the FiO2 or ventilator settings. We use indwelling arterial catheters for this purpose. To monitor trends in oxygenation continuously, we use pulse oximeters. In more stable infants, capillary blood from warmed heels may be adequate for monitoring PaCO2 and pH.
-
-
Continuous positive airway pressure
-
Indications. We begin continuous positive airway pressure (CPAP) therapy as soon as possible after birth in infants with RDS, including those at extremely low gestational age (see Chap. 29). Although both the CPAP or Intubation at Birth (COIN) and Surfactant, Positive Pressure, and Oxygenation Randomized Trial (SUPPORT) trials found that the rate of death or bronchopulmonary dysplasia (BPD) was not different between groups that received early CPAP or surfactant treatment, infants in the nasal CPAP group required less mechanical ventilation. The rate of pneumothorax was higher in the CPAP infants in the COIN trial, but not in SUPPORT trial. In infants with RDS, CPAP appears to help prevent atelectasis, thereby minimizing lung injury and preserving the functional properties of surfactant, and allowing reduction of oxygen concentration as the PaO2 rises. Infants who fail early nasal CPAP and require intubation are typically extremely immature or those with severe respiratory distress, who require FiO2 higher than 0.4 or 0.5 to maintain the targeted oxygen saturation, and have PaCO2 greater than 55 to 60 mm Hg. Infants with RDS who require intubation and mechanical ventilation should be treated with surfactant.If CPAP enables the infant to inspire on a more compliant portion of the pressure—volume curve, PaCO2 may fall. However, minute ventilation may decrease on CPAP, particularly if the distending pressure is too great. We obtain a chest radiograph before or soon after starting CPAP to confirm the diagnosis of RDS and to exclude disorders in which this type of therapy should be approached with caution, such as air leak.
-
Methods of administering CPAP. We usually begin CPAP through nasal prongs using a continuous-flow ventilator. We generally start at a pressure of 5 to 7 cm H2O, using a flow high enough to avoid rebreathing (5—10 L/minute), then adjust the pressure in increments of 1 to 2 cm H2O to a maximum of 8 cm H2O, observing the baby’s respiratory rate and effort and monitoring oxygen saturation. An orogastric tube is placed to decompress swallowed air. Simpler CPAP delivery devices utilizing tubing submerged in sterile water to deliver the desired distending pressure (“bubble” CPAP) can also be used and may have some benefits over a continuous-flow ventilator. Variable flow CPAP devices that reduce the work of breathing, especially during expiration, are available, although significant longterm clinical benefits have not been observed with their use.
-
Problems encountered with CPAP
-
CPAP may interfere with venous return to the heart and thereby decrease cardiac output. Positive pressure may be transmitted to the pulmonary vascular bed, raising pulmonary vascular resistance and thereby promoting right-to-left shunting. The risk of these phenomena increases as RDS resolves and lung compliance improves. In this circumstance, reduction of the CPAP may improve oxygenation.
-
Hypercarbia may indicate that CPAP is too high and tidal volume is reduced.
-
The use of nasal prongs may be unsuccessful if crying or mouth opening prevents adequate transmission of pressure or if the infant’s abdomen becomes distended despite insertion of an orogastric tube. In these situations, endotracheal intubation may be necessary.
-
-
Weaning. As the infant improves, we reduce the FiO2 in decrements of 0.05 to maintain the targeted oxygen saturation. Generally, when FiO2 is <0.30, CPAP can be reduced to 5 cm H2O, monitoring oxygen saturation. Physical examination will provide evidence of respiratory effort during weaning, and chest radiographs may help estimate lung volume. Lowering of the distending pressure should be attempted with caution if lung volume appears low and alveolar atelectasis persists. We generally discontinue CPAP if there is no distress and if the FiO2 remains <0.3.
-
-
Surfactant replacement
Stay updated, free articles. Join our Telegram channel
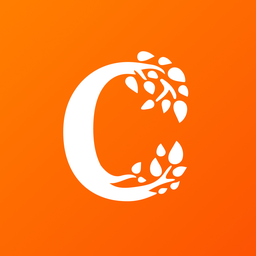
Full access? Get Clinical Tree
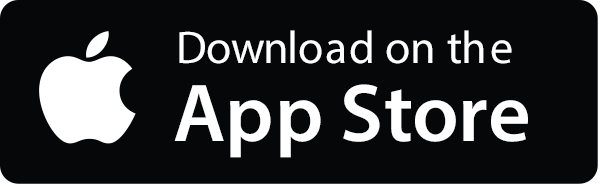
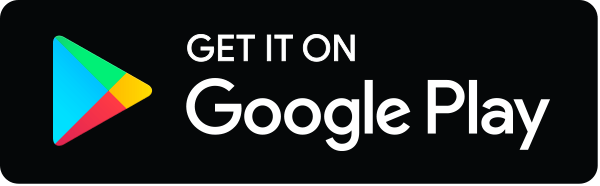