Key points
- •
Virtually all human diseases have an underlying genetic component. All health care professionals need a basic level of familiarity with genetics and genomics because it is an integral part of mainstream medicine.
- •
Genetic variation between healthy individuals exists in the form of single nucleotide polymorphisms (SNPs) and copy number variants (CNVs) providing different “doses” of a large repeated DNA sequence at a given genetic locus or of alterations in three-dimensional DNA structures called epigenetic modification.
- •
Naturally occurring variations in genetic sequence, such as SNPs, may mediate an individual’s susceptibility to disease or responsiveness to a particular medication.
- •
The human genome contains about 3 billion base pairs, of which only about 1.5% makes up the exome, or protein-coding portion. The remaining 98.5% of the human genetic sequence encodes biologically active nucleic acid molecules, which are an active area of molecular biology research.
- •
Genetic pathology can arise from alteration in the sequence of a gene, changes in the normal amount of a gene product, or sequence changes in regulatory regions that prevent the cell from expressing the intended gene product. The old term mutation has been replaced by variant, which is further classified as benign, likely benign, of unknown significance, likely pathogenic, or pathogenic.
- •
When a heterozygous individual who has an autosomal dominant trait mates with a normal individual, 50% of their offspring will have the trait.
- •
When two individuals who carry an autosomal recessive trait mate, 25% of their offspring will have the trait and 50% will be carriers.
- •
X-linked recessive characteristics are transmitted from maternal carriers to male offspring and will affect 50% of the male offspring.
- •
The mechanism of trinucleotide repeat disorders is DNA misalignment during meiosis, which leads to unstable dynamic expansion of the number of three-nucleotide repeats within the gene as it is passed from generation to generation, causing progressively more severe manifestations of disease.
- •
Prader-Willi and Angelman syndromes demonstrate the concept that certain regions of genetic material are imprinted and depend on dose and inheritance from two separate gametes for normal function.
- •
The phenotype and severity of disorders that affect the mitochondria are determined by the source of the genetic material (nuclear or mitochondrial DNA), the proportion of affected mitochondria inherited by the cell, and the threshold for energy production needed in the affected cell.
- •
In general, if a couple produces an offspring with a multifactorial defect and the problem has never occurred in the family, it can be expected to be repeated in 2% to 5% of subsequent pregnancies.
- •
Individuals who carry balanced rearrangements of genetic material are phenotypically normal, but their gametes are at risk for unbalanced genetic content, which may lead to infertility or recurrent pregnancy loss.
- •
Nondisjunctional events have been described in every autosome except chromosomes 1 and 17. Live births can result from nondisjunctional events involving chromosomes 21, 18, 13, or 22, and these occur more commonly with advancing maternal age.
- •
Screening and diagnostic tests are available for prenatal diagnosis of a large number of genetic diseases and syndromes. Indications for testing may include family history of a specific disease or recurrent clinical phenotype, ethnic background with increased carrier frequency of a certain disease, poor obstetric outcome history, increased maternal age, or population-based prevalence risk.
- •
In genetic counseling the role of the care providers (genetic counselors and physicians) is to conduct nondirectional counseling of screening and diagnostic options to provide prospective parents with information to optimize pregnancy outcomes based on their personal values and preferences. Before screening or testing, the patient needs to understand the options that may result from a positive test result.
- •
If an individual proceeds with carrier screening and is found to be a carrier of an autosomal recessive genetic condition, screening should be offered to his or her reproductive partner to assess the risk of disease in any of their children. Screening should also be offered to family members of the known carrier, who may also be an increased risk of carrying the same variant.
- •
Current recommendations state that all women who are considering pregnancy or are currently pregnant should be offered carrier screening for cystic fibrosis and spinal muscular atrophy, regardless of family history or reported ethnic background.
- •
Genetic screening based on cell-free DNA may be pursued by any patient regardless of risk.
- •
Definitive diagnosis of a genetic disease in the prenatal period requires tissue diagnosis obtained through invasive testing methods such as preimplantation genetic diagnosis, chorionic villus sampling, or amniocentesis.
- •
Aneuploidy screening and confirmatory prenatal diagnostic testing should still be offered to all women with pregnancies achieved via in vitro fertilization with preimplantation genetic testing because testing does not eliminate risk of genetic disorders and is not 100% accurate.
- •
With next-generation sequencing, it is possible to determine the complete sequence data for an individual exome (protein-coding region) or genome (complete genetic material). However, accurate interpretation of these data is limited by unknown functions of natural genetic variation and noncoding DNA regions, which make up the majority of the sequence.
- •
All cancer is genetic. However, most cancer is not inherited. For a normal cell line to be transformed into a malignant cell line, several genetic pathogenic variants in that somatic cell line must occur that alter cell growth and differentiation.
- •
In 5% to 10% of families with a history of cancer, a germline (inherited) pathogenic variant is present that predisposes certain tissues to become malignant.
- •
Types of genes and genetic mechanisms involved in malignancy may be grouped into four categories: oncogenes, tumor suppressor genes, DNA repair genes, and epigenetic mechanisms of aberrant DNA packaging.
- •
Oncogenes (gain of function) behave as growth-promoting genes, and they act in a genetically dominant manner. In other words, only one abnormal copy of the gene is needed to produce a clinically relevant phenotype because of increased gene function.
- •
Tumor suppressor genes restrain cell growth in damaged cells; therefore loss of the tumor suppressor gene through introduction of a pathogenic variant leads to increased cell proliferation of abnormal cells and thus to cancer development. They account for the majority of autosomal dominant cancer syndromes.
- •
DNA repair genes identify and mend DNA replication errors made during replication. When they are nonfunctional, replication errors can lead to cancer development.
- •
Epigenetic mechanisms involve alterations in patterns of DNA methylation or DNA packaging (3D structure determined by histone interaction and nucleosome positioning).
- •
Lynch syndrome has a prevalence of 3% to 5% in all patients with newly diagnosed endometrial cancer.
- •
In patients with endometrial cancer, the actual tumor tissue can be screened for Lynch syndrome using immunohistochemical analysis for the four mismatch repair proteins (MLH1, MSH2, MSH6, and PMS2), microsatellite instability analysis, and MLH1 hypermethylation testing.
Genetic basis of disease
Medical research and medical care have been profoundly influenced by the advancement of science that resulted in sequencing the human genome, allowing scientists to concentrate on translating this genomic text to meaningful prose. As the code is deciphered with increasing resolution, it is apparent that virtually all human diseases have an underlying genetic component, although the conversion from genotype to ultimate clinical phenotype is not always easily understood .
The overarching goals of medical care have not changed: diagnose, treat, and focus on disease prevention. The new promise of medicine in the postgenomic era is to individualize these goals, such that lifestyle interventions, screening modalities, and pharmaceuticals ultimately can be tailored to each person based on his or her unique genomic sequence. These goals have begun to materialize through examples such as detailed breast cancer screening for women in families with known BRCA1 or BRCA2 variants and tailored chemotherapeutic regimens based on molecular testing of an individual tumor. Furthermore, there is unprecedented public accessibility to the genomic screening technology and application of genetic information to medical treatment. Since completion of the Human Genome Project in April 2003, technology has advanced at an extraordinary pace to allow high-throughput data generation at an increasingly reasonable cost. High-throughput methods involve automation of experiments or assays to allow for simultaneous large-scale repetition. Over the first postgenomic decade, the time to prepare and sequence a complete human genome plummeted from 13 years to a matter of 3 to 4 days, and the cost dropped from a bit less than $30 million to around $1000 ( ).
As a result, genetics is a field with which all health care professionals, not just subspecialists, need a basic level of familiarity. Genetics, genomics, and the technology to interpret the information are now integral parts of mainstream medicine ( Table 2.1 ). The obstetrician/gynecologist is often the first-line provider helping patients navigate this complicated landscape. This chapter focuses on developing a basic understanding of genetic makeup, heritability, and the most commonly used tools for detecting genetic disorders in patients and their offspring.
G eneral R eference | ||
National Human Genome Research Institute (NHGRI) | www.genome.gov | |
S equence D atabases | ||
GenBank: Collection of all publicly available DNA sequences | National Institutes of Health (NIH) | www.ncbi.nlm.nih.gov/genbank |
Genome Reference Consortium: Maintains responsibility for the human reference genome | Members: The Genome Center at Washington University, Wellcome Trust Sanger Institute, European Bioinformatics Institute (EBI), and National Center for Biotechnology Information (NCBI) | www.ncbi.nlm.nih.gov/grc |
SNPedia: Wiki investigating human genetics | River Road Bio, LLC ( ) | www.SNPedia.com |
ISGR: The International Genome Sample Resource | EMBL-EBI and the Wellcome Trust: Maintain and build upon the 1000 genomes reference data, the largest public catalogue of human variation and genotype data | www.1000genomes.org |
ENCyclopedia Of DNA Elements (ENCODE) | International consortium to annotate functional elements in the genome ( ) | www.encodeproject.org |
Database of Genomic Structural Variation (dbVar) | NCBI database of human genomic structural variation | www.ncbi.nlm.nih.gov/dbvar |
G enotype /P henotype C orrelation | ||
Database of Genotypes and Phenotypes (dbGaP): Description and results of studies investigating interaction of genotype and phenotype | NCBI archive and distribution center | www.ncbi.nlm.nih.gov/gap/ |
Online Mendelian Inheritance in Man (OMIM) | McKusick-Nathans Institute of Genetic Medicine, Johns Hopkins University School of Medicine ( ) | omim.org |
Genome-Wide Association Study (GWAS) Central | ( ) | www.gwascentral.org |
G enome B rowsers | ||
Ensembl | Wellcome Trust, Sanger Institute, European Bioinformatics Institute | www.ensembl.org |
University of California Santa Cruz (UCSC) Genome Bioinformatics | University of California at Santa Cruz | genome.ucsc.edu |
NCBI | National Center for Biotechnology Information | www.ncbi.nlm.nih.gov/genome |
Building blocks of genetics
Molecular building blocks
Genetic information is encoded in deoxyribonucleic acid (DNA) in the nucleus of each cell of the body. DNA molecules are made up of two complementary linear sequences of nucleotides intertwined together as a double helix. The backbone of the linear DNA molecule is composed of a phosphate and a pentose sugar (deoxyribose) to which is attached a nitrogen base. Four such bases are found in a DNA molecule: two purines (adenine [A] and guanine [G]) and two pyrimidines (thymine [T] and cytosine [C]). Purine and pyrimidine occur in equal amounts; A is always paired with T in the two strands of the double helix, and G is always paired with C. The order of bases along the molecule is the genetic sequence , and the complete sequence of all 6 billion bases in an individual cell nucleus (3 billion paired bases, arranged in linear antisense strands) makes up the human genome .
The Central Dogma published by Francis Crick in 1970 remains at the heart of molecular biology ( ). The DNA is transcribed to a complementary ribonucleic acid (RNA) molecule (messenger RNA), which may be modified by regulatory sequences or three-dimensional (3D) structure. Three-base codons are read and translated to amino acids, which are linked to form a protein with some function within the cell or organism ( Fig. 2.1 ). The traditional concept of a gene refers to a unit of DNA sequence that codes for production of a protein. Surprisingly, with completion of the Human Genome Project, this gene-centric view of biology turned out to be only the tip of the iceberg in understanding the complex manner in which the genetic sequence translates to human life. Of the 3 billion base pairs that make up the genome, only about 1.5% of the assembled sequence codes for proteins. This coding portion, or exome contains about 20,000 to 25,000 genes, which is only a fraction of previous estimates that were predicated based on gene numbers correlating with complexity of the species ( ). There is now significant interest in the remaining 98.5% of the genome and how it carries out the blueprint of life. There is a growing field of discovery in the regulatory function of specialized noncoding RNA molecules, called microRNA (miRNA) , which appear to be the gatekeepers of many biologic processes ( ).

Mitosis/meiosis
The full genome consists of two copies of the total DNA sequence, packaged into two homologous sets of 23 separate chromosomes (22 autosome pairs and 1 allosome, or sex chromosome pair). During cell division, an exact replica of this biologic blueprint is passed to each daughter cell through the process of mitosis. The formation of gametes requires even distribution of the chromosomes to the progeny through the process of meiosis, as described in Chapter 1 . On fertilization, the zygote regains a full diploid complement of genetic material, equally derived from each parent. This process of replicating, packaging, and passing on genetic material from generation to generation forms the basis of heredity. Furthermore, errors in these processes can cause sequence changes or rearrangement of larger portions of DNA, introducing genetic variation or pathology, depending on the location of the change.
Genomic variation
Genetic variation
On April 14, 2003, the Human Genome Project was declared complete, with successful sequencing of the full human genome. Initial interpretation of the sequence result claimed 99.9% similarity between healthy individuals at the DNA sequence level, leaving only 0.1% of the genome sequence to account for individual differences in phenotype ( ). Each alternative form of genetic code at any given locus is referred to as an allele. An individual inherits two alleles of every genetic locus, one from each parent. If both inherited alleles have the same sequence, the individual is homozygous for the given locus. If the alleles are different, the individual is heterozygous . The allelic options at any given genetic locus derive from single nucleotide substitutions within the DNA sequence. Population sampling has demonstrated that among healthy individuals, the genetic sequence differs at around 10 million points (out of 3.2 billion DNA base pairs). These naturally occurring differences are called single nucleotide polymorphisms , or SNPs. To be classified as an SNP, two or more versions of nucleotide sequence must be present in at least 1% of the general population. The term SNP is used to describe genetic variation of healthy individuals because no disease-causing nucleotide change is this common. An example of an SNP known to mediate susceptibility to disease is the delta 32 allele of the beta-chemokine receptor 5, or CCR5. Individuals carrying one copy of the delta 32 allele are somewhat resistant to infection by human immunodeficiency virus (HIV), the virus that causes acquired immunodeficiency syndrome (AIDS), and individuals with two copies (delta 32 homozygotes, ∼1% of the white population) are almost completely immune to infection by HIV ( ). Thus the genetic variant is not the cause of disease (HIV) but is importantly associated with the manifestation of disease in humans.
In addition to individual sequence variation, comparative genome studies between individual sequences have revealed a far more pervasive form of genetic variation, termed copy number variants (CNVs) ( ; ). These are structural variants, made up of relatively large DNA segments (ranging in size from 1000 base pairs [bp] to 500,000 bp or more) that appear in a variable number at a given genetic locus and cumulatively affect 360 million nucleotides, or about 12% of the human genome ( ). A CNV can be either benign or pathogenic, and a large proportion of identified CNVs have as yet unknown significance.
Thus although SNPs introduce genetic variation at the level of individual base substitutions, CNVs represent variation in the “dose” of a relatively large DNA segment. The collection of genetic sequence variants (SNPs) or CNVs within an individual forms a sort of biologic landscape that will influence how that person experiences or responds to external influences such as challenge from an invading pathogen or ultraviolet ray exposure from the sun. Therefore understanding genetic variation in the form of SNPs and CNVs and their biologic influence can reveal a predisposition toward disease, variable susceptibility to infections, or diverse responses to pharmacologic agents as well as side effects from the same compounds. In other words, genetic variation is at the core of our collective goal of “individualized medicine,” in which preventive strategies or “designer drugs” can be tailored to individuals based on one’s genomic information.
Epigenetic variation
There are forms of genetic variation that do not involve a change in nucleotide sequence. Instead, persistent alterations in three-dimensional DNA structure can change the expression pattern of a gene. Covalent modification of histones to alter chromatin structure and the covalent addition of methyl groups to cytosine residues in the DNA are the most common three-dimensional DNA alterations. These patterns of epigenetic modification of genes are replicated through successive cell divisions despite unchanged DNA sequence and have the potential to be heritable ( ).
Two well-studied mechanisms of epigenetic modification influencing disease phenotype include genomic imprinting and CpG island methylation patterns. Genomic imprinting is a process by which hypermethylation of a specific parental allele causes that allele of the gene to be silenced, and disease may arise if the remaining allele is abnormal. Variable methylation of CpG islands in cancer cells can promote tumorigenesis through loss of proliferative supervision of the cell. Epigenetics is a growing field enhancing our understanding of complex genotype-phenotype interactions. Epigenetic mechanisms such as methylation have been shown to play a role in multiple other human disease types beyond cancer, including neurodevelopmental disorders, neurodegenerative and neurologic diseases, and autoimmune diseases ( ).
Genetic pathology
High-frequency sequence variants, CNV, and epigenetic modifications are part of the normal backdrop of the human genome, providing variation within the species without negative consequences. Overt genetic pathologic change, in contrast, usually arises from genetic variants that disrupt the normal expression or function of one or more genes. The old term mutation has been replaced by variant, which is further classified as benign, likely benign, of unknown significance, likely pathogenic, or pathogenic. The latter two categories refer to changes in the genetic code that lead to altered function and clinical consequences based on consistent human case reports, identification of the type of variant, and verification by functional studies and/or animal models. The variant may involve changing a single base or a larger segment, in which bases are removed, duplicated, or inserted. Variants occur as a result of environmental damage to DNA, through errors during DNA replication or repair, and through uneven crossing over and genetic exchange during meiosis. The loss or gain of bases in a protein-coding region may disrupt the reading frame of the triplet codons. Alternatively, a change in base sequence in a noncoding region of DNA may alter the ability of regulatory proteins or RNA molecules to bind to the DNA. Variants within the gene could result in an amino acid substitution, leading to different products with altered functions. Fig. 2.2 demonstrates such an occurrence for sickle cell anemia, which is caused by the substitution of a single base at a single point. In contrast to sickle cell anemia, for which there is only one variant in one gene, more than 1000 variants or alleles have been described to date for the cystic fibrosis transmembrane conductance regulator (CFTR) gene. Some genes also have regions that are more at risk for variant-producing events (hot spots).

Single gene disorders
Disease-causing genetic alterations are categorized by patterns of familial segregation. Any evaluation of the segregation pattern of a trait or disease in a family requires the development of a three-generation pedigree ( Fig. 2.3 ). This graphic representation of family history data assists in determining the transmission pattern of the gene and predicting the risk of recurrence. In some conditions the pattern of transmission and the constellation of clinical characteristics of affected individuals in the pedigree provide the diagnosis, which otherwise would not be evident if only one individual were evaluated.

Mendelian inheritance patterns
Autosomal dominant
In an autosomal dominant mode of inheritance, only one copy of the variant gene is required for expression of the trait, and the individual is said to be heterozygous for the trait. There are more than 4000 known autosomal dominant conditions, and most occur in the heterozygous form in affected individuals. With a few exceptions, autosomal dominant conditions occurring in the homozygous form (two copies of the affected gene) are rare, the phenotype is more severe, and the conditions are often lethal. An example is achondroplasia, in which two copies of the variant gene result in a lethal condition.
The general characteristics of autosomal dominant inheritance are illustrated in Fig. 2.4 and summarized as follows:
- 1.
Every affected individual has an affected parent (unless this is a new pathogenic variant—to be discussed later). The inheritance pattern is vertical.
- 2.
If reproductively fit, the affected person has a 50% risk of transmitting the gene with each pregnancy.
- 3.
The sexes are affected equally.
- 4.
There is father-to-son transmission.
- 5.
An individual who does not carry the pathogenic variant will have no risk of transmission to his or her offspring.

Three additional properties associated with, but not exclusive to, autosomal dominant traits are variable expressivity, penetrance, and new pathogenic variants. Variable expressivity describes the severity of the phenotype in individuals who have the pathogenic variant. Some autosomal dominant conditions have a clear clinical demarcation between affected and unaffected individuals. However, some conditions express the clinical consequences of the pathogenic variant in varying degrees among members of the same family and between different families. These differences in expression are modified by age, the sex of the affected individual, the individual’s genetic background, and the environment. Variable expression of a condition can lead to difficulties in diagnosis and interpretation of inheritance pattern. Penetrance refers to the probability that a gene will have any clinical manifestation at all in a person known to have the pathogenic variant. A condition is 100% penetrant if all individuals with the pathogenic variant have any clinical feature of the disease (no matter how minor). A number of autosomal dominant conditions are the result of new pathogenic variants. For example, about 70% of achondroplasia cases occur as new variants. Because this condition has 100% penetrance, the recurrence risk in subsequent pregnancies in the normal parents of an affected child is extremely low, but the risk to the offspring of the affected is 50%. If an autosomal dominant condition is associated with poor reproductive fitness, then the likelihood that the cases occurred because of a new pathogenic variant is greater.
Autosomal recessive
Autosomal recessive conditions are rare and require the affected individual to have two copies of the pathogenic variant allele (homozygous) to manifest the condition. In the heterozygote carrier, the product of the normal allele is generally able to compensate for the pathogenic variant allele and prevent occurrence of the disease. Fig. 2.5 is a typical pedigree illustrating autosomal recessive inheritance. The following general statements can be made about an autosomal recessive trait:
- 1.
The characteristic will occur equally in both sexes.
- 2.
For an offspring to be at risk, both parents must have at least one copy of the pathogenic variant.
- 3.
If both parents are heterozygous (carriers) for the condition, on average 25% of the offspring will be homozygous for the pathogenic variant and will manifest the condition and 50% will be carriers and will be unaffected. The remaining 25% will not have inherited the variant at all, will be unaffected, and will not be at risk of transmitting the variant to any offspring.
- 4.
Consanguinity is often present in families demonstrating rare autosomal recessive conditions.
- 5.
If the disease is relatively rare, it will be clustered among the siblings and will not be seen among other family members such as ancestors, cousins, aunts, and uncles.

Because autosomal recessive conditions require two copies of the pathogenic variant allele, and because most matings are not consanguineous, counseling couples about the risk for an autosomal recessive condition requires knowledge of the carrier frequency of the condition in the general population . Cystic fibrosis exemplifies the importance of knowing the population in which screening or counseling is being provided ( Table 2.2 ). Depending on the ethnic group of the mother and father, the risk for a child having cystic fibrosis could be as high as 1 in 1936 (1/22 × 1/22 × 1/4) if they are of Northern European descent or considerably less so if they are of Asian descent.
Ethnicity | Chance of Being Carrier | Chance Both Carriers * |
---|---|---|
European descent | 1 in 29 | 1 in 841 |
Hispanic American | 1 in 46 | 1 in 2116 |
African American | 1 in 65 | 1 in 4225 |
Asian American | 1 in 90 | 1 in 8100 |
* The chance of an affected child being born to these couples is the chance that both are carriers multiplied by 0.25.
X-linked trait
The human X chromosome is quite large, containing about 160 million bps, or about 5% of the nuclear DNA. Of the 500 genes that have been mapped to the X chromosome, 70% are known to be associated with disease phenotypes. Diseases caused by genes on the X chromosome are said to be X linked, and most are recessive. In contrast, the Y chromosome is quite small, about 70 million bps, and contains only a few genes.
The expression of genes located on the X chromosome demonstrate a unique characteristic known as dosage compensation, a concept that was described by Mary Lyon in the 1960s to explain the equalization of X-linked gene products in men and women ( ). Achievement of dosage compensation is through the principles of X inactivation, also known as the Lyon hypothesis . The tenets of the Lyon hypothesis are as follows:
- 1.
One X chromosome in each cell is randomly inactivated in the early female embryo (soon after fertilization).
- 2.
The inactivation process is random: Either the paternally or maternally derived X chromosome is chosen. The woman is thus a mosaic for genes located on the X chromosome.
- 3.
All descendants of the cell will have the same inactive X chromosome.
The Lyon hypothesis is supported by clinical evidence derived from animal and human observations of traits located on the X chromosome, such as the calico cat pattern of red and black patches of fur on female cats but not on male cats. In humans, men and women have equal quantities of the enzyme glucose-6-phosphate dehydrogenase (G6PD), which is encoded by a gene on the X chromosome. The mechanism for X inactivation is unknown at this time but clearly requires the presence of the X inactivation center, which has been mapped to the proximal end of the long arm of the X chromosome (Xq). This center contains an unusual gene called the X-inactive specific transcript (XIST), which seems to control X inactivation, a process that cannot occur in its absence .
The principles of the Lyon hypothesis remain true for the majority of genes located on the X chromosome. The silencing of these genes appears to occur as a function of DNA methylation at the promoter regions of these genes. However, several regions remain genetically active on both chromosomes. They include the pseudoautosomal regions located at the tips of the long and short arms , which are the regions that contain the genes for steroid sulfatase, the Xg blood group, and Kallmann syndrome (hypogonadism and anosmia). The pseudoautosomal region on the short arm shares extensive homology with the Y chromosome and is the region involved in the pairing of the X and Y chromosome at meiosis.
Another exception to the Lyon hypothesis is that one X chromosome is nonrandomly, preferentially inactivated. This is observed for most cases of translocations between an X chromosome and an autosome. If the translocation is balanced, the structurally normal X chromosome is preferentially inactivated. If the translocation is unbalanced, then the structurally normal X chromosome is always active. These nonrandom patterns of inactivation functionally minimize the clinical consequences of the chromosomal rearrangement. Studies can be done to look at patterns of inactivation, as in the case of prenatal diagnosis, to predict the clinical consequences of a de novo X/autosome translocation in the fetus.
Random inactivation confers a mosaic state for the female carrier. The normal allele is able to compensate for the abnormal allele (as in autosomal recessive traits), and female carriers of X-linked recessive conditions usually do not have clinical manifestations of the disease. Occasionally, however, there is a skewed, or less than 50/50, chance of inactivation such that the X chromosome carrying the normal allele is inactivated more frequently. In such cases, female carriers display some features of the condition and are referred to as manifesting heterozygotes . Manifesting heterozygotes have been described for hemophilia A, Duchenne muscular dystrophy, ornithine transcarbamylase deficiency, and X-linked color blindness. Genetic counseling of recurrence risks for an X-linked recessive condition depends on the sex of the affected parent and of the offspring. Fig. 2.6 is a pedigree illustrating X-linked recessive inheritance, the characteristics of which are the following:
- 1.
Affected individuals are usually male unless X-chromosome activation is skewed in the female carrier or the individual is homozygous for the trait.
- 2.
The affected men in a kindred are related through women.
- 3.
The gene is not transmitted from father to son.
- 4.
All daughters of affected men will be carriers.
- 5.
Daughters of female carriers have a 50% chance of being carriers; sons of female carriers have a 50% chance of being affected.

X-linked dominant inheritance
The major feature of X-linked dominant inheritance is that all heterozygotes, both male and female, manifest the condition . Although the pedigree may resemble autosomal dominant inheritance, the distinguishing feature is that affected men never have affected sons, and all daughters of affected men are affected. There are usually more affected women than men, and the majority of the women are heterozygotes. Examples of diseases with this mode of inheritance are hypophosphatemic rickets and Rett syndrome.
Nonmendelian inheritance patterns (complex traits)
Trinucleotide-repeat disorders: Unstable repeated sequence variants
In the early 1990s a new class of genetic conditions was recognized as being caused by unstable dynamic variants in a gene. In classic genetic inheritance the diseases and their inheritance patterns are due to pathogenic variants that are passed on from generation to generation in a stable form. That is, all affected members in a family have the identical inherited variant. In 1991, however, a number of reports began to describe a new class of genetic condition in which the gene sequence variation was dynamic and changed with different affected individuals within a family. The most common group of disorders is known as triplet, or trinucleotide repeat, disorders. More than a dozen diseases are now known to be associated with unstable trinucleotide repeats ( Table 2.3 ) ( ).
Repeat Number | ||||||
---|---|---|---|---|---|---|
Disease | Inheritance Pattern | Triplet Repeat | Location of Expansion | Normal | Unstable | Affected |
Huntington disease | Autosomal dominant | CAG | Exon coding region | <36 | 29-35 | >35 |
Fragile X | X-linked | CGG | 5′ untranslated region | <45 * | 56-200 | >200 |
Myotonic dystrophy | Autosomal dominant | GTG | 3′ untranslated region | <35 | 50-100 | >100 |
Spinal cerebellar ataxias † | Autosomal dominant | CAG | Exon | <40 | Different for each subtype | >40 |
Friedrich ataxia | Autosomal recessive | GAA | Intron of gene | <33 | 34-65 | >65 |
* Fragile X intermediate mutations (45-54 CGG repeats) may expand to premutations but not full mutations
† Spinal cerebellar ataxias are a heterogeneous group of conditions, all of which appear to be associated with a CAG repeat. Each subtype has its own specific range of normal, unstable, and affected repeat sizes.
These conditions are characterized by an expansion of variable size, within the affected gene, of a segment of DNA that contains a repeat of three nucleotides such as CAGCAGCAG (CAG)n, or CCGCCG (CCG)n. These triplet repeats are unstable in that they tend to expand as the gene is passed on from generation to generation. The molecular mechanism is most likely misalignment at the time of meiosis. The result of increasing triplet expansion is progressively earlier onset and/or more severe manifestation of disease with each successive generation. This phenomenon is known as anticipation.
The commonality of this group of genetic conditions stops at the shared molecular mechanism. Each disease, otherwise, has its own features. Some, such as myotonic dystrophy, are inherited in an autosomal dominant pattern, but others, such as Friedrich ataxia, are autosomal recessive conditions. The susceptibility of the triplet repeat to expansion also may depend on the parent of origin: It is typically more severe with paternal inheritance in Huntington disease and exclusively maternal in fragile X syndrome.
Fragile X syndrome
Fragile X syndrome, a disease within the unstable triplet repeat disorder group, is the most common heritable form of moderate intellectual disabilities and is second to Down syndrome among causes of intellectual disabilities in men. The affected gene (fragile X mental retardation 1, FMR1 ) is located on the X chromosome at Xq27.3, and triplet repeat expansion in the gene causes a pattern of abnormalities, including intellectual disabilities, characteristic facial features, and autistic behaviors. Disease frequency is approximately 1 in 4000 men and 1 in 8000 women. The condition is due to an expansion of the triplet repeat CGG located in the untranslated region of the first exon of the gene. The triplet expansion blocks normal function of the FMR1 gene, thus causing the syndrome. Fragile X is associated with maternal anticipation, meaning female carriers are at increased risk of passing on expanded repeated sequence variants to their children, whereas the repeated sequence variants in male carriers will not typically expand when passed on to their daughters . Risk of expansion may also be associated with the original size of the repeated sequence variant and the presence or absence of AGG repeats within the CGG expanded region ( ).
Individuals with normal X chromosome alleles have CGG repeats in the 6 to 44 range, whereas affected individuals have expanded “full mutations” of 200 to more than 1000 repeats. Individuals with 55 to 200 repeats are known as premutation carriers. Men are more likely to be affected with fragile X than women, and features of the condition tend to be milder in women than men. Individuals with 45 to 54 CGG repeats are known as intermediate carriers. Intermediate carriers are not at an increased chance to have children affected with fragile X syndrome. However, female intermediate carriers are at an increased chance to have children who are premutation carriers (14% risk of expansion). Premutation carriers are not usually affected with features of fragile X syndrome, but they are at increased risk for having affected children or descendants if the premutation expands to a full mutation in successive generations. Conversely, intermediate and premutation alleles can be passed on without expanding, and in rare instances, expanded alleles may even contract when inherited.
Long-term follow-up of premutation carriers has revealed that these individuals are at increased risk for certain adult-onset conditions separate from the fragile X syndrome. Male premutation carriers are at increased risk for fragile X–associated tremor/ataxia syndrome (FXTAS) later in life ( ). FXTAS may also occur in female premutation carriers, though more rarely. Female premutation carriers are also at increased risk for premature ovarian insufficiency (POI), which is the cessation of menses before age 40. In contrast, intermediate carriers are not at any increased risk to develop FXTAS or POI ( ).
Although the unstable triplet is transmitted in an X-linked pattern, the probabilities of the different phenotypes are far from traditional X-linked inheritance. Understanding the features of fragile X syndrome is crucial for genetic counseling and assessment of recurrence risks. The possible outcomes of the offspring of a premutation female carrier are the following:
- 1.
Male offspring—three possibilities:
- a.
Unaffected: does not inherit the X chromosome with the premutation.
- b.
Unaffected: inherits the X chromosome with the premutation that did not expand. At risk for FXTAS and at risk for passing the premutation to daughters, who in turn will be at risk for having children who are premutation carriers or affected.
- c.
Affected: inherits an expanded full mutation.
- a.
- 2.
Female offspring—four possibilities:
- a.
Unaffected: does not inherit the X chromosome with the premutation.
- b.
Unaffected: inherits the X chromosome with the premutation that did not expand to a full mutation. At risk for POI and FXTAS and at risk for having children who are premutation carriers or affected.
- c.
Unaffected: inherits the X chromosome with an expanded full mutation; about 50% of women with a full mutation appear to be clinically unaffected.
- d.
Affected: inherits the X chromosome with an expanded full mutation.
- a.
Genomic imprinting and uniparental disomy
Genomic imprinting and uniparental disomy refers to the differential activation or expression of genes depending on the parent of origin. In contrast to Mendel’s hypothesis that the phenotype of a gene is no different if inherited from the mother or the father, we now understand that there is a group of diseases in which the parent of origin of a gene or chromosome plays a role in the phenotype of the affected individual. The best-studied examples of this mechanism are Prader-Willi syndrome (PWS) and Angelman syndrome (AS). Both diseases arise from loss of function of the same gene on chromosome 15, but two different disease phenotypes arise depending on which parental allele is affected. PWS is characterized by obesity, hyperphagia, small hands and feet, hypogonadism, and mental retardation ( ). In about 70% of cases, cytogenetic deletion of the proximal arm of the paternally inherited chromosome 15 is observable (15q11-q13). In contrast, the same deletion of the maternally inherited chromosome 15 results in the Angelman phenotype of severe mental retardation, short stature, spasticity, and seizures ( ). Interestingly, 30% of subjects with PWS do not have a cytogenetic deletion but rather inherit two intact chromosomes 15 from the mother. No genetic information on chromosome 15 is inherited from the father. This is referred to as maternal uniparental disomy. Individuals with Angelman syndrome without a cytogenetic deletion have two copies of the paternally derived chromosome 15 and no chromosome 15 from the mother, a condition termed paternal uniparental disomy . These findings indicate that for the region of 15q11-q13, the expression of the PWS phenotype is brought on by the absence of a paternal contribution of the genes in this region. Likewise, the expression of Angelman syndrome is due to the absence of the maternal contribution of genes located at 15q11-q13. The genes in this region are said to be “imprinted” because their parent of origin has been “marked.”
Many regions of the human genome have now demonstrated evidence of imprinting. Knowledge of diseases that occur as a result of imprinting are important for clinical care because they have implications for prenatal diagnosis, especially when mosaicism is encountered.
Germline mosaicism
Mosaicism is defined as the presence of two or more genetically different cell lines in the same individual or in tissue derived from a single zygote. All women, because of X inactivation, are mosaics for genes on the X chromosome. Mosaicism, however, is not necessarily evenly or randomly distributed throughout the body. In other words, using the entire body as the whole organism, an individual is mosaic either because different organs or tissues have genetically different cells but each organ or tissue has the same cell line or because the genetically different cell lines are dispersed throughout many tissues in the body. The distinction between these two types of mosaicism is particularly important in making a prenatal diagnosis in cases in which mosaicism is identified in amniotic fluid cells. For instance, one cannot be confident that a fetus identified as having trisomy 21 mosaicism would necessarily have a less severe mental retardation phenotype because of mosaicism. The brain cells could potentially all contain full trisomy 21, but the cells of the skin could all be normal diploid. In germline mosaicism, the implication is that the pathogenic variant is present in only one parent and arose during embryogenesis in all or some of the germ line cells but few or none of the somatic cells of the embryo. This concept was developed to explain recurrence of a genetic condition in a sibship (usually autosomal dominant) in which incorrect diagnosis, autosomal recessive inheritance, reduced penetrance, or variable expression could not be the reason for the recurrence. The best example of germline mosaicism is osteogenesis imperfecta type II (lethal form). At the molecular level, the pathogenic variant causing the condition is dominant—that is, only one copy of the abnormal gene is necessary to cause this perinatal lethal condition. Yet there are families in which multiple affected pregnancies are seen in the same couple or one parent has recurrences with different partners. If the spontaneous pathogenic variant rate for an autosomal dominant variant is 1 chance in 10 5 , then the probability of two independent spontaneous variants for the same lethal autosomal dominant condition is (1/10 5 ) 2 , a highly unlikely event. Germline mosaicism is now well documented for about 6% of cases of osteogenesis imperfecta type II ( ). Unfortunately, the exact recurrence risk in an individual family is difficult to assess because the proportion of gametes containing the pathogenic variant is unknowable.
Mitochondrial inheritance: Maternal inheritance
Most inherited conditions occur as a result of variants in the DNA of the nucleus (nuclear genome). However, mitochondria have their own DNA molecules, which contain a small fraction of genes whose products are vital to the function of the cell. Mitochondrial DNA (mtDNA), which was completely sequenced in 1981, is small, about 16.5 kilobase pairs (kb), and is packaged as a circular chromosome located in the mitochondria. A growing number of conditions resulting from abnormalities of the mitochondria have now been identified. Because the mitochondrial apparatus and its function are under the control of both nuclear and mitochondrial genes, many diseases affecting the mitochondria do not follow the typical Mendelian pattern of inheritance. Each human cell contains a population of several hundred or more mitochondria in its cytoplasm. Most of the subunits that make up the mitochondrial apparatus are encoded by the nuclear genome.
Because the primary function of the mitochondria is to provide energy for the cell in the form of adenosine triphosphate (ATP), genetic variants that affect the genes that code for oxidative phosphorylation will likely result in cell dysfunction and death. The organs most affected would be those that depend heavily on mitochondria. The diseases that result are generally neuromuscular in nature, such as encephalopathies, myopathies, ataxias, and retinal degeneration, but the genetic variants have pleiotropic effects, meaning multiple different clinical traits are caused by a single gene defect ( ).
The most significant characteristic of mitochondrial diseases caused by pathogenic variants in mtDNA is that they are all maternally inherited . This is because the cytoplasm of the ovum is abundant with mitochondria, but the sperm contain very few mitochondria. Therefore an individual’s mitochondria (and mtDNA) are essentially all inherited from the mother. If the mother has a mtDNA pathogenic variant, then all of her children will inherit that variant. When a pathogenic variant arises in the DNA of a mitochondrion in the cytoplasm of the ovum, it begins as one variant in one mitochondrion. However, as replication and division of this variant mitochondrion occur, they become randomly distributed among the normal mitochondria and between the daughter cells. One daughter cell by chance may contain a large population of mitochondria with the pathogenic variant, but the other may have none or very little. Fertilization of the egg with a large proportion of mitochondria containing the variant would result in an offspring at risk for manifesting a mitochondrial disease. Leber hereditary optic neuropathy (LHON) is a well-known mitochondrial disease in which rapid, bilateral loss of central vision occurs as a result of a pathogenic variant in mitochondrial DNA. Men and women are affected equally, and all affected individuals are related through maternal lineage.
A second feature of mitochondrial diseases is that of variable expression. Within each cell and tissue, there is a threshold for energy production below which the cells will degenerate and die. Organ systems with large energy requirements are most susceptible to mitochondrial abnormalities. Thus if an mtDNA pathogenic variant is present, the severity of the mitochondrial disease will depend on the proportion of mitochondria with the variant that the individual inherited from his or her mother and the susceptibility of different tissues to altered ATP metabolism.
In contrast, abnormalities of mitochondrial function caused by pathogenic variants of genes encoded in the nuclear genome will exhibit traditional Mendelian inheritance patterns; autosomal dominant, autosomal recessive, and X-linked patterns of mitochondrial disorders have been observed. A few mitochondrial diseases occur as sporadic somatic pathogenic variants and have little or no recurrence risk. Table 2.4 lists some of the known diseases of mitochondrial function and their inheritance patterns.
Disease | Features | Genetics | Inheritance Pattern |
---|---|---|---|
Barth syndrome | Dilated cardiomyopathy, cyclic neutropenia, skeletal myopathy, growth deficiency, abnormal mitochondria | Nuclear DNA encoding mitochondrial protein tafazzin ( TAZ gene) | X linked |
Friedreich ataxia | Limb movement abnormalities, dysarthria, absent tendon reflexes | Nuclear DNA encoding mitochondrial protein frataxin ( FXN gene, triplet repeat) | Autosomal recessive |
Leber hereditary optic neuropathy (LHON) | Blindness, rapid optic nerve death in young adulthood | Mitochondrial DNA | Maternal |
Leigh disease (subacute necrotizing encephalomyelopathy) | Infant-onset progressive psychomotor regression after viral illness, hypotonia, peripheral neuropathy, lactic acidosis | Many genes: 20%-25% mitochondrial DNA (includes neuropathy, ataxia, and retinitis pigmentosa [NARP]) | Mitochondrial DNA: maternal |
75%-80% nuclear DNA | Nuclear DNA: autosomal recessive or X linked | ||
MERRF | Myotonic epilepsy, ragged red fibers in muscle, ataxia, sensorineural deafness | Mitochondrial DNA | Maternal |
MELAS | Mitochondrial encephalopathy, lactic acidosis, strokelike episodes, sensorineural deafness | Mitochondrial DNA | Maternal |
MIDD | Maternally inherited diabetes and deafness | Mitochondrial DNA | Maternal |
MNGIE | Mitochondrial neurogastrointestinal encephalopathy, childhood-onset gastrointestinal dysmotility, peripheral neuropathy | Nuclear DNA ( TYMP gene) causes destabilization of mitochondrial DNA | Autosomal recessive |
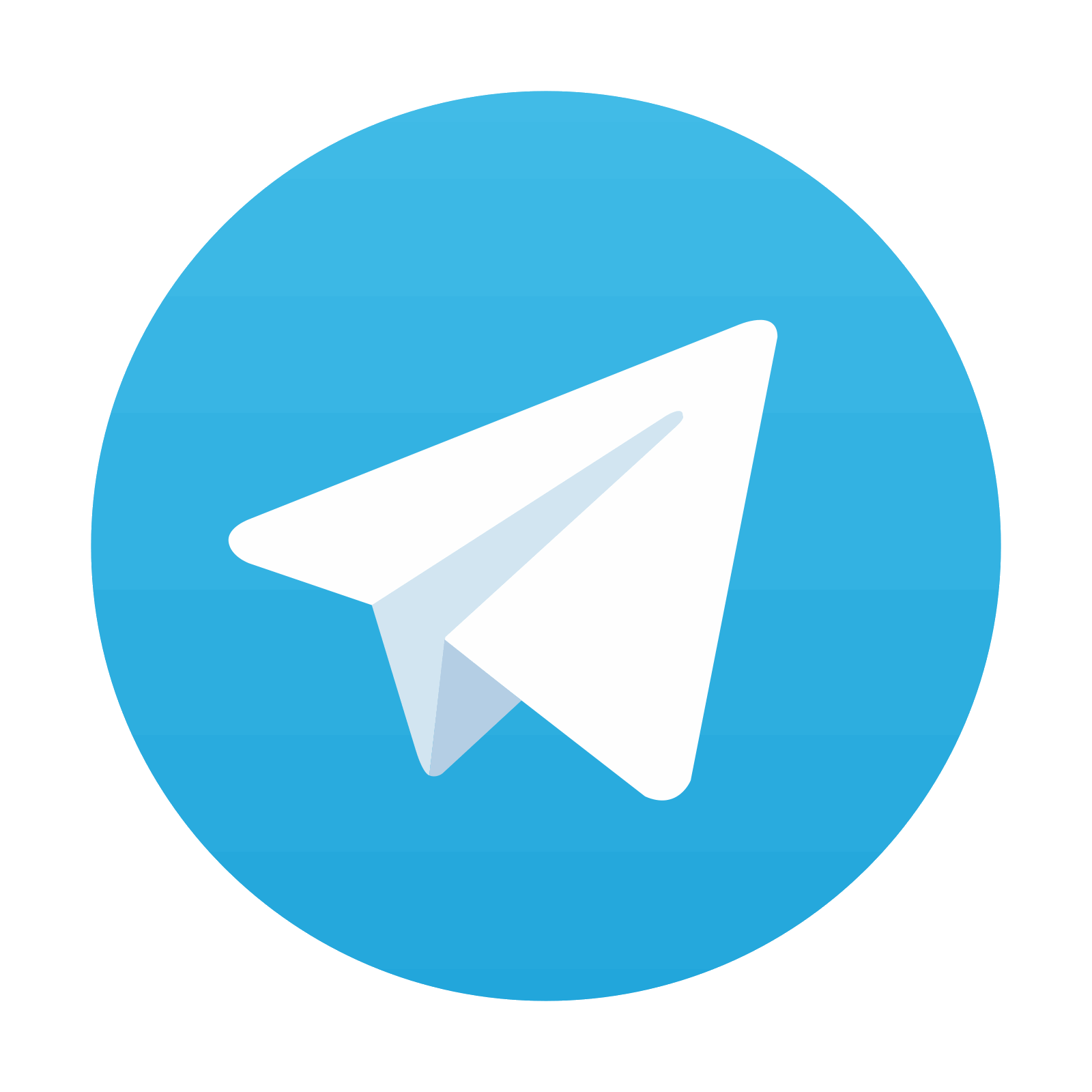
Stay updated, free articles. Join our Telegram channel
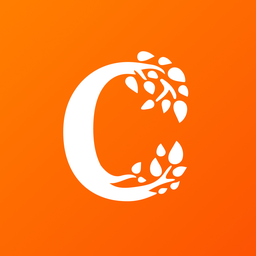
Full access? Get Clinical Tree
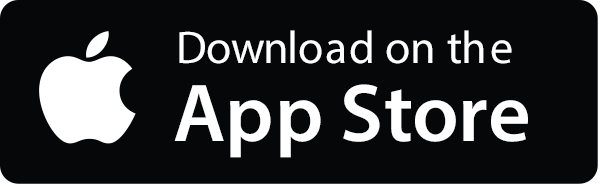
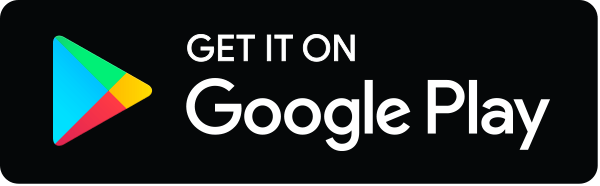
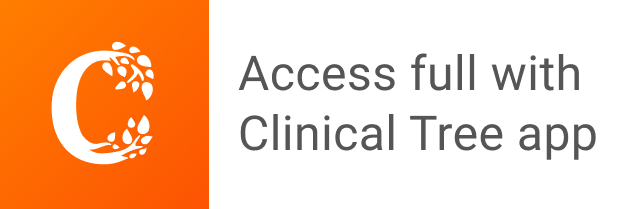