1. RS alone to exploit the biology of large single doses of radiation in low-grade tumors
2. RS as an adjunct to more conventionally fractionated radiation to escalate tumor dose
3. RS to treat recurrent tumors after prior radiation
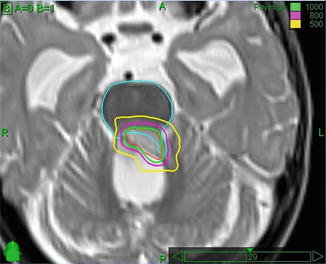
Fig. 18.1
Radiosurgery boost after conventional intensity-modulated radiotherapy for an ependymoma. Note the dose avoidance of the brainstem. The 1,000, 800, and 500 cGy isodose lines are shown
Evidence to support the use of RS in the pediatric population is limited [5, 6], and no comparative trials can be expected in the small heterogeneous patient population of children with small, well-defined tumors amenable to radiosurgery. Although published results are generally favorable, RS carries a small risk of symptomatic radiation necrosis. The anti-VEGF antibody bevacizumab may be a new option for selected cases of necrosis refractory to steroids [7].
Special Techniques
Craniospinal Irradiation
One of the most complex and time- and resource-consuming techniques performed in radiotherapy departments, craniospinal irradiation (CSI), is made easier through the use of CT simulation combined with modern treatment planning and delivery.
Patient Positioning and Immobilization
CSI has traditionally been delivered to patients in the prone position, but most modern techniques are designed with treatment in the supine position. The supine position is more comfortable and, if anesthesia is required, safer because of better airway control (Fig. 18.2). Immobilization is essential but technique specific. While use of a thermoplastic mask is routine, full-body immobilization devices are variably used now, with emphasis shifting from immobilization to daily volumetric image guidance (e.g., using Tomotherapy®).
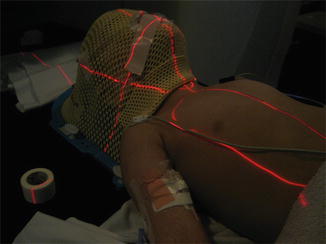
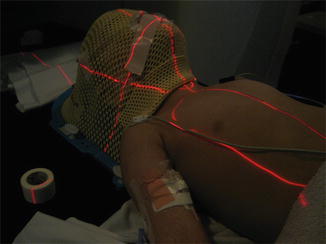
Fig. 18.2
A 3-year-old patient being treated under anesthesia for craniospinal irradiation. Note the thermoplastic immobilization device fixed to the treatment couch
Target Volume Definition
The CTV for CSI consists of the whole brain and spinal cord and the overlying meninges. Careful target volume definition is critical, both to avoid recurrence in underdosed areas and to reduce the risk of late effects. For the brain, thin-slice CT simulation is essential to ensure adequate coverage of the CTV in the subfrontal region. Co-registration of a postoperative T2-weighted MRI to the planning CT is helpful in ensuring coverage of CSF along lower cranial nerve roots and in delineating the lateral aspect of CTV for the spine field that includes the extension of the meninges along the nerve roots to the lateral aspects of the spinal ganglia. A T1-weighted sequence usually better identifies the caudal limit of the target volume which could be higher or lower than the traditional lower border for the spine field of the bottom of the S2 foramina.
Treatment Planning and Delivery
With traditional 2D CSI planning technique based on bony anatomy, large volumes of normal nontarget tissues are exposed to radiation. The move to CT simulation and 3D planning has reduced the field size in most patients. The use of IMRT results in further reductions of dose to structures directly anterior to the spine at the expense of increased low-dose exposure [8]. Further improvements to both can be achieved using daily image guidance that permits the use of smaller PTV margins.
There are many issues that need to be addressed in designing a CSI technique, most especially field matching in the cervical region and dose homogeneity throughout the target volume. Using modern tools for treatment planning and delivery, it is possible to greatly simplify the technique and substantially reduce planning and delivery times (Fig. 18.3a) [9]. In general, when posterior beams are used, 6 MV photons provide satisfactory target coverage. A variation of dose along the spinal axis of greater than 10 % will require the use of dose compensation that can be achieved using multileaf collimation. Helical tomotherapy techniques further simplify planning and treatment by avoiding the need for junctions and improving conformality to the target (Fig. 18.3b) [10].
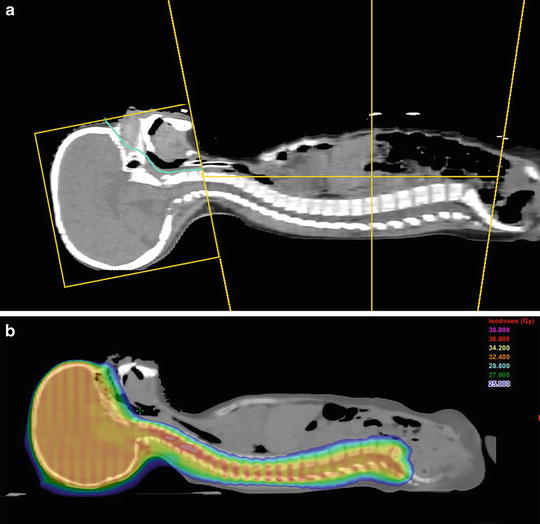
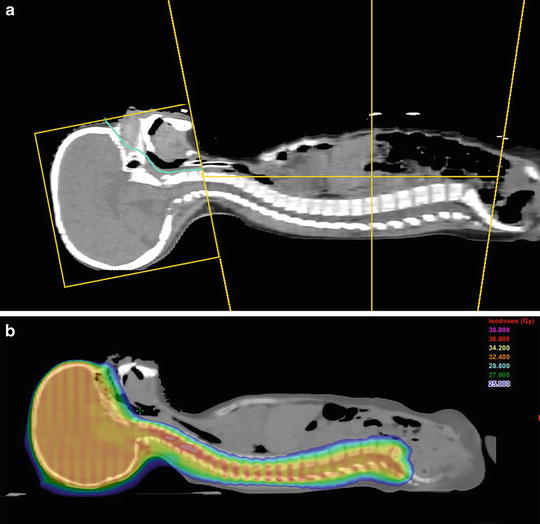
Fig. 18.3
(a): In a 3D CSI technique, lateral opposed fields are used to treat the brain and a direct posterior field is used to cover the spinal axis. The field junction over the cervical cord usually is moved weekly (“feathered”) to avoid over- or underdosage. The supine position is more comfortable for the patient and safer if sedation or anesthesia is required. In the technique shown, fixed field parameters are used which greatly facilitates treatment planning and delivery (Parker IJROBP 2006). (b): An example of an IMRT technique using TomoTherapy®. The absence of junctions is a major advantage of this technique. Note the excellent conformality to the target volume and significant high-dose sparing of structures anterior to the target (Parker IJROBP 2010)
Proton Therapy
Protons interact with matter differently from the high-energy X-rays routinely used in radiotherapy. A proton beam deposits a large proportion of its energy over a range of a few millimeters in the so-called Bragg peak with no exit dose. Although the entrance dose is increased when the Bragg peak is spread out to cover the target volume, the integral dose is still substantially reduced in comparison with photon beams (typically by a factor of 1.5–3). In pediatric radiotherapy, it is this reduction in integral dose as well as sparing of OARs from low and intermediate radiation doses that motivates the use of protons. The dose to OARs can be reduced quite dramatically, the most striking example being that of craniospinal radiation where exit dose to the thyroid, breasts, heart, and liver can be completely eliminated. In a Swedish planning study of craniospinal radiation, the lifetime risk of secondary cancer was estimated at 30 % for intensity-modulated photons as compared with only 4 % for intensity-modulated protons [11]. In a planning study of optic glioma, craniopharyngioma, and medulloblastoma, a dose-cognitive effect model predicted higher IQ scores and/or academic reading scores following proton treatment [12]. In other situations, long-term benefits may be expected from dose sparing of OARs such as the pituitary gland and hypothalamus, the optic apparatus, and cochleae.
Although protons have been used to treat cancer since 1954, the availability of the modality has been very limited and few children have been treated. As an example, a 2004 report from the Loma Linda University Medical Center reported on the first three patients treated with proton craniospinal radiation (from 2001 to 2003) [13]. With such small and relatively recent series, few clinical data are available to support the benefits predicted by dose models. A comparison of patients of all ages treated in Boston from 1974 with matched photon patients from the SEER database has been published in abstract form [14]. At a median follow-up of more than 6 years, the crude rate of second malignancies was 6.4 % for proton patients compared with 12.8 % for photon patients. Of the 503 proton patients in this study, only 15 were children and none had developed secondary malignancies. As new facilities become operational and better data are collected, we are beginning to see prospective reports showing reduced acute toxicity in pediatric CNS patients, notably health-related quality of life and ototoxicity [15, 16]. However, these reports suffer from the lack of an unbiased comparable photon-treated group, and accurate estimates of late toxicity and cancer induction will require much longer follow-up.
Although very attractive, the implementation of proton therapy does have caveats. The relative biological effectiveness of protons is incompletely characterized and varies, for example, by cell line. The proton dose distributions will likely be less forgiving to contouring errors, positioning variations and intra-treatment variations in patient contours/density. The unique dosimetry also poses new clinical questions such as the effect of sharp gradients on bone growth. Despite the lack of clinical evidence, the ASTRO Emerging Technology Committee’s 2012 review of proton beam therapy noted that “the rationale for using PBT in posterior fossa tumors, optic pathway tumors, and brainstem lesions is compelling” and that “future clinical studies reporting on the outcome of patients treated with protons will decide how widespread protons become for pediatric CNS tumors” [17]. In practice, it is expected that the percentage of pediatric CNS tumors treated with proton therapy will increase yearly as new facilities come on line, confidence with the technology grows, and more clinical data become available.
Re-irradiation
Re-irradiation may be an option for some children with CNS tumors although the literature is scarce. This is partly explained by the fact that doses received by OARs during the first course of radiotherapy are typically high, approaching accepted tolerance doses. As well, pediatric oncologists may not be familiar with re-irradiation as a possible treatment option, with chemotherapy being the therapeutic strategy most often used at time of relapse. Most of the evidence thus comes from single institution experiences.
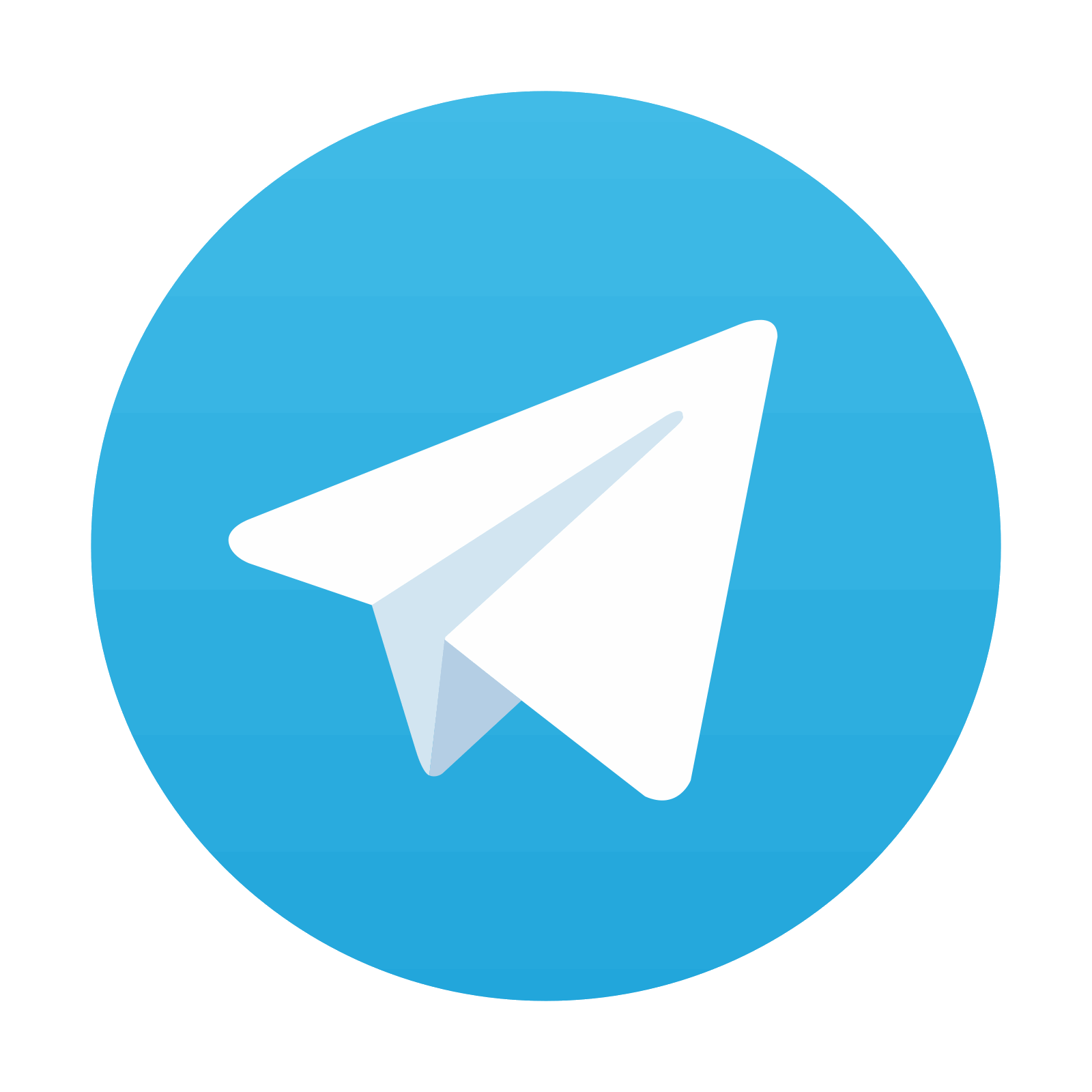
Stay updated, free articles. Join our Telegram channel
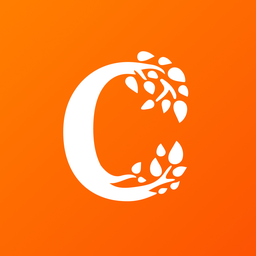
Full access? Get Clinical Tree
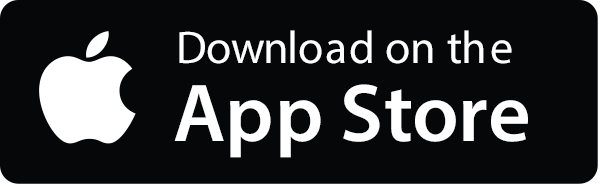
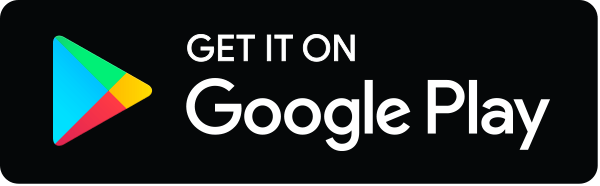