Key Points
- •
Chorionic villi, amniotic fluid and fetal blood are the specimen types currently used for prenatal diagnostic testing for chromosome abnormalities.
- •
The spectrum of chromosomal alterations seen during prenatal testing include autosomal or sex chromosome aneuploidy, balanced or unbalanced structural rearrangements, triploidy, supernumerary marker chromosomes, submicroscopic deletions and duplications, mosaicism and uniparental disomy.
- •
Methods for detecting chromosomal abnormalities in prenatal specimens include karyotype of G-banded chromosomes, fluorescence in situ hybridisation, chromosomal microarray, quantitative fluorescence polymerase chain reaction, multiplex ligation-dependent probe amplification and next-generation sequencing (NGS).
- •
Chromosomal single nucleotide polymorphism microarrays are invaluable for diagnosing clinically relevant microdeletions and microduplications. Unlike whole-chromosome aneuploidy from nondisjunction, the risk for submicroscopic copy number variants is not dependent on maternal age. With these advances in our knowledge and testing capabilities, all pregnant women should be offered prenatal diagnostic testing for chromosome abnormalities.
- •
Noninvasive prenatal screening using maternal peripheral blood is currently used to assess the risk for common trisomies, sex chromosome aneuploidies and select microdeletion syndromes. Follow-up with confirmatory diagnostic testing is recommended for all screen positive pregnancies.
- •
Challenges in the diagnosis of chromosome abnormalities include interpreting mosaicism and copy number variants with incomplete penetrance or variable expressivity, as well as the risk in de novo balanced rearrangements.
- •
Future prospects for prenatal detection of chromosome abnormalities are currently centred on NGS to improve the capabilities of noninvasive prenatal screening.
Introduction
Prenatal diagnosis of chromosome abnormalities has been in practice since the 1960s and continues to be a crucial part of prenatal care. Initially, testing was performed during the second trimester by amniocentesis. Concerns over safety of the procedure led to studies to assess increased risks to the pregnancy as a result of amniocentesis. These studies showed the increased rate for miscarriage or spontaneous abortion from amniocentesis to be approximately 1 in 200 or 0.5%. This risk was lower than the risk for a woman older than the age of 35 years at the time of delivery having a fetus with a chromosomal abnormality by karyotype. Therefore by the 1970s the standard of care was to offer these women amniocentesis. Advances in prenatal testing continued through the 1980s with the advent of chorionic villus sampling (CVS), a procedure that could be performed in the first trimester, offering families prenatal diagnosis earlier in the pregnancy. Cohort studies have shown that gestational timing is important to reduce the risk for limb defects from CVS, which occur when the procedure is done at less than 8 weeks. The standard of care is to perform CVS between 10 and 12 weeks’ gestation. CVS may carry a slightly higher risk for miscarriage compared with amniocentesis, but the risk from either procedure is significantly lower than the original studies suggested. Recently, a large meta-analysis found the risk for procedure-induced miscarriage to be approximately 1 in 450 for CVS and 1 in 900 for amniocentesis.
Historically, prenatal testing had been considered in the context of large genomic imbalances involving whole chromosomes or large chromosomal regions that could be identified by classical cytogenetic analysis. Molecular cytogenetic techniques have improved prenatal chromosome diagnostic capabilities to include the detection of microdeletions and microduplications that are not discernable by standard cytogenetic analysis. These newer techniques include fluorescence in situ hybridisation (FISH), quantitative fluorescence polymerase chain reaction (QF-PCR), multiplex ligation-dependent probe amplification (MLPA) and chromosomal microarray, with each offering unique advantages. The reduced risk for adverse outcomes from CVS and amniocentesis coupled with modern advances in the diagnosis of genomic imbalance now provide safer, more comprehensive testing to any woman interested in prenatal diagnosis.
Prenatal Specimens
Prenatal specimens are primarily obtained by CVS or amniocentesis. In some instances, percutaneous umbilical blood sampling (PUBS) is performed, usually as a follow-up to a previous procedure that yielded ambiguous results. These different methods are used during different stages of the pregnancy, and each has its advantages and limitations. All specimens can be tested using classic and molecular cytogenetic techniques to identify chromosome abnormalities in a fetus.
Chorionic Villus Sampling
Placental villi are composed of multiple layers, including the chorionic membrane that makes up the outermost layer of fetal tissue and forms the villi for vascularisation of the placenta. In CVS, villus tissue is removed under the guidance of ultrasound by either the transcervical or transabdominal method. Whereas the transcervical method involves inserting a catheter through the vagina and cervix, the transabdominal method uses a thin needle similar to an amniocentesis. The position of the uterus and placenta dictate which method is used. A small sample of chorionic villi is retrieved and sent for cytogenetic analysis. Two preparations types are possible for a CVS sample, direct and cultured. Direct preparation analyses the rapidly dividing trophoblast cells of the placenta and may produce results in 24 to 48 hours. Cultured preparations examine the mesenchymal cells from the villi and typically require 7 to 10 days for results. Cultured cells usually produce better karyotype preparations and are the preferred approach for many laboratories, although some may set up both direct and culture. DNA can be extracted for chromosomal microarray from either direct or cultured cell preparations, and results are generally available in 7 to 10 days for direct and 14 to 21 days for the cultures.
Amniocentesis
The amniotic sac surrounds the fetus and holds the amniotic fluid. Amniotic fluid contains a variety of cell types both from the fetus and the amnion. Amniocentesis involves removing a sample of amniotic fluid through a thin needle inserted through the maternal abdomen and into the gestational sac under ultrasound guidance. Amniocentesis is the most widely used procedure for obtaining fetal specimens. It is most commonly performed between 15 and 17 weeks’ gestation but not before 14 weeks because of the association with talipes equinovarus and the higher risk for miscarriage. Cells are cultured in flasks and on coverslips and may take up to 1 week before there are a sufficient number of cells for cytogenetic analysis. The success rate for culture of amniocentesis samples is approximately 99%, and the reliability of the cytogenetic diagnosis is also around 99%. Early amniocentesis (performed before 14 weeks’ gestation) does carry a 1% to 2% risk for clubfeet ; therefore CVS is the preferred procedure for patients seeking early prenatal diagnosis. As with CVS, DNA can be extracted directly from the cells within the amniocentesis sample without culture and used for chromosomal microarray analysis. Results from a direct preparation are usually available in 7 to 10 days and in cultured cells in 14 to 21 days.
Percutaneous Umbilical Blood Sampling
Percutaneous umbilical blood sampling is an invasive procedure that involves obtaining a fetal blood sample. PUBS may also be referred to as cordocentesis, umbilical vein sampling and fetal blood sampling, and it was originally developed for prenatal diagnosis of blood disorders such as haemoglobinopathies. PUBS specimens are obtained through an ultrasound-guided needle inserted transabdominally into the umbilical cord at the site of insertion into the placenta, the intrahepatic portion of the umbilical vein or rarely a free loop of cord. Several studies to assess the safety of PUBS showed the procedure carried a 1% to 2% risk for fetal death or spontaneous abortion, significantly higher than CVS or amniocentesis. Recent studies have shown that the risk may be higher in fetuses with abnormalities such as nonimmune fetal hydrops or intrauterine growth restriction. For this reason, it is reserved for cases in which diagnostic information cannot be obtained through CVS, amniocentesis and ultrasound or when the results of these previous diagnostic tests were inconclusive. Occasionally, it is used in later gestations when rapid results are important for management. Unlike CVS and amniocentesis, PUBS procedures are performed after 18 weeks’ gestation. Because culture time is shorter for blood samples, results are generally available in 3 to 4 days for classic cytogenetics analysis and 7 to 10 days for chromosomal microarray.
Indications for Referral
Historically, advanced maternal age has been the most common reason for referral for prenatal diagnosis of chromosome abnormalities. Other factors that increase the risk for a fetal chromosome abnormality include positive first or second trimester aneuploidy screening, fetal ultrasound anomalies, a previous pregnancy with a chromosomal abnormality and a parent with a chromosomal rearrangement. The recent advances in molecular cytogenetics now provide a reliable way to identify additional chromosome abnormalities without an age-related risk component. Therefore the standard of care in the United States is to offer prenatal diagnostic testing to all pregnant women regardless of age.
Advanced Maternal Age
The association between increasing maternal age and Down syndrome was recognised as early as 1933 by Penrose. Advanced maternal age is generally accepted to be 35 years or older at the time of delivery. The age of 35 years was designated as the cutoff because the risk for finding a chromosome abnormality and the risk for causing a miscarriage from amniocentesis were roughly the same. Below the age of 35 years, more miscarriages could be expected compared with the number of liveborn children with a chromosome aneuploidy. The increase in fetal chromosome abnormalities in aging women is due to an increase in nondisjunction during meiosis. Fig. 24.1 shows the direct relationship between maternal age and increased risk for chromosome abnormality at the time of CVS and amniocentesis. In twin gestations, considering maternal age subgroups and twin zygosity, a significantly lower-than-expected Down syndrome incidence is seen for both monozygotic and dizygotic pregnancies than previous empiric calculations would suggest.

Advances in cytogenomic technologies such as chromosomal microarrays, noninvasive prenatal screening (NIPS), and discovery of copy number variants (CNVs) with no maternal age-related risk have led to an increasing proportion of younger women (maternal age <35 years) who are considered to be at low risk for aneuploidy to opt for prenatal cytogenetic screening and diagnostic testing.
Abnormal Screening Result
Although advanced maternal age increases the risk for fetal chromosome abnormalities, the majority of pregnancies occur in younger women because they are in their prime reproductive years. As a result, the majority of liveborn children with chromosome abnormalities are found in young mothers. To address this issue, noninvasive screening techniques were developed as a way to identify which pregnancies have an increased risk for fetal aneuploidy without incurring the risk for miscarriage from invasive CVS or amniocentesis procedures. However, because the combined incidence of fetal chromosome abnormalities (i.e. whole-chromosome aneuploidies, submicroscopic CNVs, uniparental disomy (UPD)) is higher than the risk for miscarriage from invasive procedures (0.11%–0.22%), any pregnant woman may choose diagnostic prenatal cytogenetic testing. However, most low-risk women use noninvasive screening to assess their risk before deciding whether or not to proceed with a CVS or amniocentesis. Several screening methods are currently offered to help identify pregnancies at a higher risk for fetal chromosome abnormalities.
Noninvasive Screening Using Biochemical Analytes and Assessment of Nuchal Translucency
Pregnant women have several options for noninvasive screening in both the first and second trimesters. First trimester screening involves a combination of maternal blood testing and ultrasound analysis of the fetus and can be done as early as 11 weeks. Maternal blood is tested for the levels of free β-human chorionic gonadotropin (β-hCG) and pregnancy-associated plasma protein A (PAPP-A) and combined with sonographic measurement of the nuchal translucency, the space at the back of the fetal neck. Such screening can identify 80% to 90% of fetuses with Down syndrome. Second trimester screening analyses a different set of maternal serum biomarkers. Quad screening using AFP, hCG, unconjugated estriol and inhibin A identifies approximately 67% to 76% of fetuses with Down syndrome. In all cases, the false-positive rate is around 5%. First and second trimester screening can be done as independent tests or in combination. When both first and second trimester screening tests are combined and presented as a single risk assessment report, it is called integrated screening . Sequential screening is when all screening tests are performed, but the patient may decide if the first trimester results are elevated to proceed to diagnostic testing or defer until all results are complete in the second trimester. In general, the threshold for first trimester diagnostic testing is higher (e.g. 1 in 60 to 1 in 100) than that used later (1 in 270).
Contingent screening uses first trimester screening results to guide the next steps of prenatal screening and diagnostic testing. If a pregnancy is identified as high risk using first trimester screening, it is recommended the patient consider invasive testing such as CVS or amniocentesis. If the pregnancy falls within an intermediate-risk category, the patient may proceed to second trimester screening. Finally, if the pregnancy is deemed low risk for a chromosome abnormality during first trimester screening, then no further screening would be recommended. Screening via maternal serum markers is limited to the most common chromosomal aneuploidies and has a false-positive rate of 5%. In general, screening using a combination of first and second trimester markers has a slightly higher detection rate and lower false positive rate than single trimester screening but depending on the approach chosen may delay diagnostic testing in screen positive cases.
Noninvasive Prenatal Screening Using Cell-Free Fetal DNA
Recently, the ability to analyse fetal cell-free DNA (cffDNA), present in the maternal circulation of pregnant women, has paved the way for the development of NIPS for fetal chromosomal aneuploidies (13, 18, 21 and sex chromosome anomalies) and selected microdeletions. cffDNA is placental in origin, and its absolute amount is a small proportion of the total cell-free DNA (cfDNA) component of the maternal plasma, which is a mixture of both maternal cfDNA and cffDNA (<1 μg in 20 mL of whole blood). The current NIPS technologies do not separate cffDNA from the maternal cfDNA. Analysis is performed on the entire cfDNA complement in the maternal blood without extracting or enriching the fetal fraction. NIPS relies on the use of next-generation sequencing (NGS) (whole genome or targeted) that looks for the presence of extra sequences from chromosomes of interest such as 13, 18 and 21. If the mother is phenotypically normal, the additional sequences are inferred to be derived from the fetus. Two major sequencing approaches are utilised; the first is referred to as ‘counting’ because all sequences are counted, and the DNA sequences from the chromosomes of interest are compared with a reference chromosome or chromosome set to determine whether they are in excess or missing. The second approach uses single nucleotide polymorphisms (SNPs) to assess the genotype patterns that are indicative of aneuploidy. Each of these approaches is coupled with advanced statistical algorithms and sophisticated bioinformatics software that assesses for abnormal amounts of chromosome-specific cfDNA in the maternal circulation in pregnancies with fetal aneuploidy. cffDNA is detectable in maternal plasma by 6 weeks’ gestation, increases during the pregnancy at a fetal fraction rate of 0.1%/week between 10 to 21 weeks and 1%/week after 21 weeks, and is eventually cleared from the maternal circulation within a few hours of delivery. The accuracy of any type of NIPS is limited in cases of multiple gestations, and as such, the American College of Obstetrics and Gynecology does not recommend NIPS for women with multiple gestations. Current data suggest that NIPS-based aneuploidy screening in singleton pregnancies has superior performance in screening for trisomy 21 compared with all other existing methods such as combining maternal age, first or second trimester ultrasound findings and first or second trimester serum biochemical analysis. Therefore this screening is an attractive alternative to traditional serum screening for aneuploidy. Second, compared with trisomy 21, detection rates for trisomies 18, 13 and sex chromosome aneuploidies are lower. A recent meta-analysis with the published studies on the performance of cfDNA testing in screening for aneuploidies in singleton pregnancies revealed weighted pooled detection rates and false-positive rates of 99.2% and 0.09%, respectively, for trisomy 21; 96.3% and 0.13% for trisomy 18; 91.0% and 0.13% for trisomy 13; 90.3% and 0.23% for monosomy X and 93.0%; and 0.14% for sex chromosome aneuploidies other than monosomy X. NIPS was initially validated for clinical use in high-risk patients. However, recent literature adds to its utility in the general obstetric population. It is important to note that cffDNA-based NIPS is a screening method, and given the potential for false-positive and false-negative results, it is recommended that screen-positive patients should be followed up by diagnostic testing using CVS or amniocentesis. The current limitations of NIPS include screening for only a few aneuploidies and select microdeletions, the potential for inaccurate results and a higher cost. Pregnant women opting for NIPS should be appropriately counselled about its limitation to screen for only a few aneuploidies (13, 18 and 21), about the difference between a screening test and confirmatory diagnostic testing and about the potential of NIPS to reveal incidental maternal findings such as a maternal chromosome abnormality or even uncover maternal cancer.
Abnormal Fetal Ultrasound Findings
Ultrasound is important for noninvasive monitoring of all pregnancies, from early confirmation of pregnancy to assessing fetal status through delivery. A recent meta-analysis showed an overall detection rate between 46% and 51% for fetal anomalies before 14 weeks. The detection rate in low risk or unselected populations was 32% and in high-risk groups around 60%. Neck anomalies, such as increased nuchal translucency or cystic hygroma, have the highest detection rate (92%). Abdominal defects such as omphalocele and gastroschisis are also detected at a high rate on early ultrasound. Brain, spine and heart defects had around 50% detection rate, and limb, genitourinary tract and facial defects each had only about a 34% detection rate. It is also important to note that some anomalies (e.g. agenesis of the corpus callosum, cerebellar hypoplasia, renal agenesis, duplex kidneys) will not become apparent until the second trimester and are not reliably detected on early ultrasound. Anatomy scans between 18 and 20 weeks’ gestation are the standard of care for assessing the health of fetuses, but with advances in early screening, many women choose to have an ultrasound in the first trimester.
Chromosome aneuploidies are often associated with multiple congenital abnormalities, which are often apparent on fetal ultrasonography ( Tables 24.1 and 24.2 ). In the 1990s, practitioners began using sonogram measurements of the nuchal translucency as a marker for Down syndrome. Other notable markers for chromosomal aneuploidies include cystic hygroma, nasal bone hypoplasia, echogenic intracardiac focus, exomphalos and fetal growth restriction. As with serum biomarker screening, a positive finding increases the risk but does not mean the fetus is affected with a chromosome abnormality. Generally, the more markers identified by ultrasound, the higher the risk for an affected fetus.
Chromosome abnormality | CVS | Amniocentesis | CVS + amniocentesis | ||||
---|---|---|---|---|---|---|---|
Ultrasound anomaly | All other indications | Total | Ultrasound anomaly | All other indications | Total | Overall total | |
Normal karyotype (%) | 51 | 94 | 86 | 83 | 97 | 93 | 89 |
Any chromosome abnormality (%) | 49 | 6 | 14 | 17 | 3 | 7 | 11 |
Study Total ( n ) | 411 | 1798 | 2209 | 652 | 1421 | 2073 | 4282 |
Chromosome abnormality | CVS | Amniocentesis | CVS + amniocentesis | ||||
---|---|---|---|---|---|---|---|
Ultrasound anomaly | All other indications | Total | Ultrasound anomaly | All other indications | Total | Overall total | |
Trisomy 21 (%) | 21.17 | 3 | 6.38 | 4.14 | 1.41 | 2.27 | 4.39 |
Trisomy 18 (%) | 11.19 | 0.61 | 2.58 | 5.37 | 0.07 | 1.74 | 2.17 |
Trisomy 13 (%) | 4.38 | 0.17 | 0.95 | 2.30 | 0 | 0.72 | 0.84 |
45,X (%) | 7.30 | 0.06 | 1.40 | 1.23 | 0 | 0.39 | 0.91 |
47,XXY (%) | 0.97 | 0.17 | 0.32 | 0.15 | 0 | 0.05 | 0.19 |
47,XXX (%) | 0.49 | 0.06 | 0.14 | 0 | 0.28 | 0.19 | 0.16 |
47,XYY (%) | 0 | 0.06 | 0.05 | 0.31 | 0 | 0.10 | 0.07 |
69,XXX / 69,XXY (%) | 1.7 | 0.17 | 0.45% | 1.07 | 0 | 0.34 | 0.40 |
Struct rearrangement: unbalanced (%) | 1.22 | 0.28 | 0.45% | 1.84 | 0 | 0.58 | 0.51 |
Struct rearrangement: balanced (%) | 0.24 | 1.17 | 1% | 0.31 | 1.13 | 0.87 | 0.93 |
Other nonmosaic aneuploidy a (%) | 0 | 0.17 | 0.14 | 0.15 | 0 | 0.05 | 0.09 |
Struct rearrangement: markers (%) | 0 | 0 | 0 | 0.15 | 0.14 | 0.14 | 0.07 |
Study total ( n ) | 411 | 1798 | 2209 | 652 | 1421 | 2073 | 4282 |
a Other aneuploidies include two cases of trisomy 9 and two cases of trisomy 16.
Previous Pregnancy or Child With a Chromosomal Abnormality
Pregnancy loss or a child born with a chromosome abnormality can be difficult for the family, and they often seek prenatal diagnostic testing in subsequent pregnancies. A diagnosis of triploidy, tetraploidy, 47,XYY or 45,X does not appear to increase the risk for future pregnancies to be affected by chromosome abnormalities. Cytogenetic findings with an increased recurrence risk for future aneuploidies include all nonmosaic trisomies, 47,XXY, structural rearrangements and marker chromosomes. Recurrence may be caused by advanced maternal age, as outlined earlier in this chapter. However, gonadal mosaicism in a parent and other factors associated with meiotic errors (e.g. translocation carrier) also increase the risk for subsequent pregnancies with chromosome abnormalities. It is generally accepted that the risk increases 1.6- to 1.8-fold for trisomy in future pregnancies when trisomy is found in a previous pregnancy or miscarriage. In the case of submicroscopic CNVs, the recurrence risk depends on the mode of inheritance. For de novo CNVs, the recurrence risk is similar to the general population risk. However, if a parent is a carrier, the recurrence risk increases to 50%.
Chromosome Rearrangement or Copy Number Variant in a Parent
Structural rearrangements of the chromosomes can occur in clinically normal people. These rearrangements may consist of balanced translocations (segments of two or more chromosomes are exchanged), inversions (a single segment of one chromosome is flipped) or insertions. These changes do not involve any net gain or loss of genetic material and therefore do not typically cause health issues in the carrier. However, a carrier may face difficulties with reproduction because of errors in meiotic segregation of the rearranged chromosomes. In a reciprocal translocation involving two chromosomes, the resulting gametes may contain a normal chromosome complement or the balanced translocation, leading to a clinically normal fetus. However, malsegregation can lead to partial aneuploidies where one segment of the chromosome is duplicated but the other is deleted. The net result is a partial trisomy and a partial monosomy. In general, the larger the chromosomal imbalance, the more likely the pregnancy will result in a spontaneous abortion. Pericentric inversions – those involving both arms of the chromosome – carry a similar risk for partial aneuploidies where the regions distal to the breakpoints may be duplicated or deleted. Paracentric inversions only involve a single arm of the chromosome and therefore produce gametes with normal or acentric and dicentric chromosome complements. Paracentric inversion carriers have a lower risk for birth of a child with a chromosome abnormality because the acentric and dicentric chromosomes will generally not be viable. Paracentric insertions have a higher risk for children with cytogenetic abnormalities because they may inherit unbalanced chromosomes with or without the inserted genetic material. Of note, some inversions are frequent in the population and considered normal genetic variations or polymorphisms with no known clinical consequences. One example is the pericentric inversion of chromosome 9 (inv(9)(p12q13)).
Copy number variations (CNVs) involve submicroscopic chromosome rearrangements and may be de novo or inherited. When a CNV is identified, it is important to do parental testing if their carrier status is unknown. De novo CNVs are more likely to be clinically relevant, but the recurrence risk is negligible. CNVs inherited from a clinically normal parent are more likely to represent benign, familial variants that pose no increased risk for adverse outcomes. However, there are known pathogenic CNVs that are associated with phenotypic heterogeneity because of incomplete penetrance, variable expressivity or both. One chromosomal region that is commonly involved in inherited CNVs is the short arm of chromosome 16. Deletions and duplications at 16p11.2 are associated with neurocognitive abnormalities and may be inherited from a parent who is clinically normal or only mildly affected. The recurrence risk is 50% for future pregnancies, but the clinical outcome is difficult to predict. Genetic counselling is an important aspect of prenatal diagnosis as we learn more about genetic conditions and the nuances of genotype–phenotype correlations.
There are many indications for referral for prenatal cytogenetic diagnosis, and it is important to highlight the need for genetic counselling. The risk of a fetal chromosome abnormality will vary widely depending on the patient’s previous history and current reason for referral. Genetic counsellors can integrate important clinical information and communicate with the patient so that the full scope of the inherent risk, screening results and prenatal diagnostic results are understood. This information is crucial for the patient as she makes decisions for her current and future pregnancies.
Incidence and Spectrum of Chromosomal Abnormalities in the Prenatal Setting
The incidence and spectrum of chromosome abnormalities observed in the prenatal period is highly influenced by the indication for referral as well as the gestational age at which prenatal diagnosis is performed. The likelihood of a chromosome abnormality is significantly increased when a fetal structural anomaly is detected. Additional data extraction from the 2012 National Institute of Child Health and Human Development (NICHD) microarray study indicated that karyotype abnormalities were present in 49% and 17% of fetuses with an ultrasound anomaly detected at the time of CVS and amniocentesis, respectively (see Table 24.1 ). Karyotype abnormalities were seen in 6% and 3% of CVS and amniocentesis samples, respectively, referred for all other indications (see Table 24.1 ). In centres in which ultrasound anomalies account for roughly 25% of referrals, the chances of finding a karyotype abnormality at the time of prenatal diagnosis (CVS or amniocentesis) for all indications is just over 10% (see Table 24.1 ). Table 24.2 shows the full incidence and spectrum of karyotype abnormalities observed in the 2012 NICHD microarray study.
Aneuploidy
The term aneuploidy is used when a cell has too many (>46) or too few (<46) chromosomes. The gain or loss of one or several chromosomes typically involves thousands of genes, which results in substantial genomic imbalance. Consequently, the majority of aneuploid conceptions are nonviable. Chromosome abnormalities are observed in approximately 60% to 70% of spontaneous abortions, and approximately 82% to 85% of them are aneuploidies. Thus only a few autosomal trisomies and sex chromosomes aneuploidies are routinely encountered in the prenatal setting. The most common autosomal aneuploidies are trisomy 21 (Down syndrome), trisomy 18 (Edward syndrome) and trisomy 13 (Patau syndrome). Trisomy 21 is the most frequently observed aneuploidy in prenatal diagnosis and accounts for approximately one fifth of fetuses with ultrasound anomalies at the time of CVS and only about one 25th of fetuses with structural defects at the time of amniocentesis (see Table 24.2 ). Rapid laboratory methods of detecting these common aneuploidies within 24 to 48 hours include FISH, QF-PCR and MLPA. These techniques are discussed in greater detail in the section covering diagnostic tests for chromosome abnormalities. Table 24.2 shows the incidence of these common aneuploidies at the time of CVS and amniocentesis and further stratifies their frequency according to referral indication.
The sex chromosome aneuploidies include 47,XXY (Klinefelter syndrome), 47,XXX (triple X syndrome), 45,X (Turner syndrome) and 47,XYY. The 45,X and 47,XYY chromosome complements are associated with paternal meiotic error, and the other aneuploidies are associated with nondisjunction events caused by advanced maternal age. Monosomy X is more likely to be observed in association with specific fetal ultrasound anomalies such as cystic hygroma and large nuchal translucency compared with the other sex chromosome abnormalities. In the 2012 NICHD study, 7.3% of fetuses with an ultrasound anomaly (most often enlarged NT) had monosomy X at the time of CVS (see Table 24.2 ). This number is significantly reduced to just over 1% at the time of amniocentesis (see Table 24.2 ). The sex chromosome aneuploidies can be detected by karyotype analysis and by some of the rapid testing methods described later.
Fetuses with any of these chromosomal aneuploidies can survive to term; however, spontaneous abortion is a more likely outcome. Consequently, there are a greater number of abnormalities seen at the time of CVS, and this number decreases in prevalence as the pregnancy progresses (see Fig. 24.1 and Table 24.1 ). Estimates show about 30% of trisomy 21 pregnancies ascertained at the time of CVS abort spontaneously before term (see Table 24.2 ). Similarly, 24% of trisomy 21 pregnancies at the time of amniocentesis abort before term. The phenotypes for the autosomal trisomies are well documented, making genetic counselling in these situations fairly straightforward.
Sex chromosome abnormalities usually do not present with severe clinical features or malformations. This is because of the phenomenon of X inactivation, whereby in normal females, one X chromosome undergoes a process of inactivation called lyonisation, which ‘switches off’ most of the genes. However, some X chromosome genes escape inactivation and are therefore present as two active copies in normal females. These genes include those in the ‘pseudoautosomal regions’ ( PAR1 and PAR2 , at the end of the short and long arms, respectively, of the sex chromosomes). Outside PAR1 and PAR2, about 15% of genes on the X chromosome escape inactivation, and the abnormal copy number of these genes is very likely the cause of the abnormal phenotype found in individuals with sex chromosome aneuploidy. Some patients present with learning difficulties and slightly lower IQs compared with their siblings. Infertility may be another complicating factor, depending on the aneuploidy. Medical management using hormones is beneficial in some cases, and assisted reproductive technology can be helpful in some cases with fertility issues, although pregnancies in woman with a 45,X karyotype have significant maternal complications.
Triploidy and Hydatidiform Moles
Triploidy occurs in 1% to 3% of human conceptions, although most of these are spontaneously aborted in early pregnancy (6%–7% of early spontaneous abortions). Triploidy is highly correlated with the presence of structural fetal defects and accounts for 1% to 2% of prenatal cases with structural anomalies (see Table 24.2 ). Triploidy can be accurately identified by karyotype analysis, QF-PCR and SNP microarray analysis but cannot be detected by MLPA analysis or array comparative genomic hybridisation microarrays that do not contain SNPs.
Complete triploidy
The normal human chromosome complement is diploid, in which there are two sets of chromosomes—one inherited from the mother and the other from the father. In triploidy, the extra set of chromosomes may originate from either the mother (digyny) or the father (diandry); data indicate that the phenotype of the embryo can be correlated with the parent of origin of the extra set of chromosomes and that the differences in phenotypes secondary to the parent of origin of the extra set of chromosomes may be related to imprinting in the placenta. Whereas digynic triploidy arises from the failure of the oocyte to expel the second polar body at fertilisation, diandric triploidy is thought to be caused by dispermic fertilisation, although it may occasionally arise from fertilisation with a diploid sperm. The diandric triploid state may result in a partial molar pregnancy, but if this is avoided, the diandric or digynic triploid fetus may survive well into pregnancy, although few survive until term, and no nonmosaic live-born child is known to have survived the neonatal period.
Mosaic triploidy
Triploidy may occur in mosaic form; mechanisms for mosaic triploidy include fusion of two zygotes, one normal and one triploid, to give a chimeric fetus; delayed fertilisation of a zygote with a second sperm; and reincorporation of the second polar body into the fertilised egg. Mosaic triploidy may be viable to term, depending on the proportion and distribution of the two cell lines. A case has been reported of mosaic triploidy detected at CVS, in which the triploid cell line was only present in extraembryonic tissues; the pregnancy resulted in a normal infant.
Complete hydatidiform moles
These pregnancies are diploid, but both sets of chromosomes are derived from the father. Most are caused by fertilisation of an anucleate egg by a single sperm followed by doubling of the paternal chromosomes; very rarely, molar pregnancies arise after fertilisation of an anucleate egg by two different sperm. Molar pregnancies are generally sporadic in their aetiology; however, recurrence in families has been documented, and in some cases, biparental inheritance has been demonstrated. This is thought to be caused by mutation of a gene involved in the pathway leading to the ‘reprogramming’ of imprinted genes in the maternal germline, resulting in gametes with paternally imprinted chromosomes. Complete molar pregnancies have an associated risk for choriocarcinoma. Confirmation of genome-wide uniparental paternal disomy in complete hydatidiform moles that are diploid is possible by chromosomal microarray analysis that includes SNPs.
Partial hydatidiform moles
Some diandric triploid conceptuses result in partial molar pregnancies. These are not thought to be associated with any risk for choriocarcinoma. A partial molar pregnancy has been described with triploidy mosaicism, in which the triploid cell line was confined to the placenta but the diploid fetus survived to term.
Partial Aneuploidy Caused by Structural Rearrangements and Copy Number Variants
In contrast to whole-chromosome aneuploidies, partial aneuploidy involves a smaller number of genes and a greater likelihood of survival to birth. The size of the imbalance and gene content are important for understanding the likelihood of abnormal clinical features and overall prognosis. A G-banding pattern on karyotype and FISH studies was traditionally used to identify the nature of partial aneuploidies. Advances in chromosomal or cytogenomic microarray analysis (CMA) now provide a faster, more accurate assessment of the origin and nature of chromosome imbalances. Partial aneuploidies may result when an unbalanced rearrangement is inherited from a parent with a balanced translocation or inversion. Deletions, duplications, supernumerary marker chromosomes, ring chromosomes and isochromosomes also result in partial aneuploidies.
When a partial aneuploidy is identified in the fetus, parental chromosome studies are required to determine whether the variant is de novo or familial in nature. In general, unbalanced, de novo changes are more often associated with adverse outcomes. De novo and familial rearrangements that are balanced are more often associated with a favourable prognosis. Classical cytogenetic studies have shown that about 94% of de novo apparently balanced rearrangements are not associated with adverse clinical outcomes, but 6% are. Such apparently balanced rearrangements can occur in about 0.4% of pregnancies. Refinements of the risk for an adverse outcome in cases ascertained with an apparently balanced rearrangement have become possible using microarray and NGS technologies. In cases with clinical sequelae, CMA and NGS have identified submicroscopic imbalances and additional structural complexity that were not discerned at the resolution of the standard G-banded karyotype. In contrast, unbalanced rearrangements are more often associated with congenital anomalies and other adverse outcomes regardless of whether they are de novo or inherited from a balanced parent. CNVs, like other structural imbalances, are more likely to be deleterious if they are de novo and benign if inherited from a phenotypically normal parent. However, caution must be used in the interpretation of certain CNVs identified by microarray analysis. As more submicroscopic balances are identified, it is apparent that a proportion of them will be associated with phenotypic heterogeneity. Therefore the offspring may be severely affected even when the parent carries the same CNV and is clinically normal. Counselling must take into account the type of imbalance and inheritance and discuss the risk for subsequent pregnancies if the parents were not previously known to carry a structural rearrangement or CNV.
Long Contiguous Stretches of Homozygosity
Chromosomal microarray analysis has advanced prenatal diagnostics by providing a method to detect submicroscopic chromosomal abnormalities. Copy number changes as small as a few kilobases are detectable by copy number oligonucleotide probes, but SNP oligonucleotide probes are necessary to determine whether a region is heterozygous or homozygous. Long contiguous stretches of homozygosity (LCSH) observed in multiple chromosomes are suggestive of consanguinity (identity by descent) in the parents. There is an increased risk for autosomal recessive diseases in these LCSH regions because the genes within these regions are now homozygous. Noting the regions of homozygosity can be helpful in identifying candidate genes for further diagnostic testing and expanded carrier testing of the parents is recommended.
In some cases, two copies of a chromosome may be inherited from a single parent, a phenomenon called UPD. In UPD, there is no aneuploidy per se, but the fetus is homozygous along the entire length of a chromosome ( Fig. 24.2 and Table 24.3 ). Similar to cases of consanguinity, the finding of UPD (two identical chromosomes) leads to an increased risk for autosomal recessive diseases in genes in the homozygous region. If the parent is a heterozygous carrier of a deleterious allele and both copies of the gene are inherited from that parent, the offspring can be affected. UPD can also cause aberrant expression of genes that are imprinted (only expressed from one parent’s chromosome and silenced on the other). Certain chromosomes contain imprinted regions (chromosomes 6, 7, 11, 14, 15 and 20) and UPD for these chromosomes can result in clinical abnormalities ( Table 24.4 ). For example, UPD for chromosome 15 can lead to Prader-Willi syndrome if both chromosomes are inherited from the mother or Angelman syndrome if both chromosomes are inherited from the father.
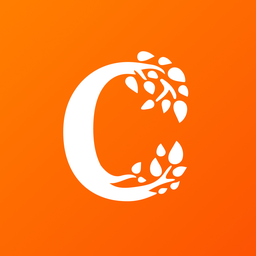
Full access? Get Clinical Tree
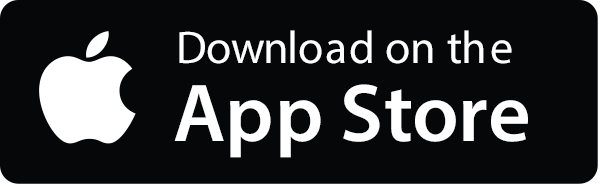
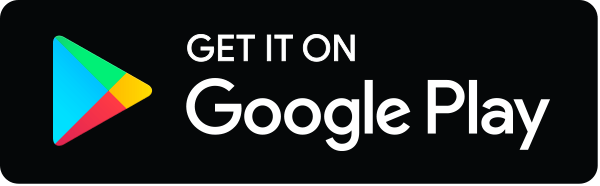