-
Chapter Contents
-
Composition of infusates 321
-
Techniques 324
-
Administration 325
-
-
Hazards 325
-
Transition to enteral nutrition 326
-
Conclusion 326
The fetus is nourished parenterally during pregnancy via the placenta. This is abruptly discontinued at birth and the vast majority of babies then make a successful transition to enteral feeding. Parenteral nutrition (PN) is indicated in the infant for whom feeding via the enteral route is impossible, inadequate or hazardous, because of malformation, disease or immaturity. Babies with congenital anomalies of the gut require PN until normal gut function has returned. In babies with necrotising enterocolitis or a chylothorax, resting the gastrointestinal (GI) tract for a prolonged period may be curative, and some babies with short gut require total or partial parenteral feeding for many months to allow gut adaptation. PN is essential for extremely preterm babies prior to enteral feeding or to supplement milk feeds, which can then be increased slowly while continuing to satisfy nutritional requirements.
The long-term impact of inadequate nutrition during the neonatal period is now becoming clearer. The third trimester is a critical period for neuronal development. Work in the rodent model has demonstrated permanent impairment in dendritic arborisation and axonal myelination if early nutrition is inadequate ( ). Clinical trials of enteral nutrition in preterm human infants have suggested that nutrition during the same period may have an impact on long-term neurodevelopmental outcome ( ). Postnatal growth failure in preterm infants is associated with an increased incidence of cerebral palsy, developmental delay and abnormal neurology ( ). It is common in our neonatal units despite widespread use of PN ( ). A significant proportion of this postnatal growth failure occurs in the first few weeks of life, with subsequent inability to meet the demands of catch-up growth on top of the requirements for normal growth ( ). A recent observation study was carried out on a random sample of all hospital patients in the UK who were given PN. Neonatal cases from a total of 74 hospitals providing all levels of neonatal care were reviewed. This revealed wide variation in practice with significant delays in starting PN and inadequate nutritional content in approximately 40% of cases reviewed ( ). As PN is an expensive and potentially hazardous intervention ( Table 17.1 ), it is important that it is prescribed carefully and effectively.
Metabolic |
|
Line-related |
Infection |
|
General |
|
Composition of infusates
Fluids (see Ch. 18 )
Preterm infants adapt poorly to inadequate or excessive fluid intake compared with term infants. They have increased amounts of extracellular fluid, their kidneys have poor concentrating and diluting ability ( Ch. 18 ), they have a large surface area in relation to weight and their insensible water loss through the skin, especially in the first week, is high ( Ch. 15 ).
Energy
The estimation of energy requirement takes into account the components of total heat production (basal metabolic rate, physical activity, specific dynamic action of food, thermoregulatory heat production) and the energy cost of growth. In preterm infants, the basal metabolic rate is about 40 kcal/kg/day ( ). The energy cost of activity is 4 kcal/kg/day with minimal handling ( ) and the specific dynamic action of PN is 13% of the basal heat production or 10% of the calories infused ( ). If a baby is nursed in a thermoneutral environment, an input of 50 kcal/kg/day is generally sufficient to match ongoing expenditure but it does not meet additional requirements of growth (see Ch. 16 ) ( ).
Growth failure will result unless additional energy is provided. The energy cost of gaining 1 g of new tissue is 5 kcal ( ). To achieve the equivalent of third-trimester intrauterine weight gain of 14–15 g/kg/day, an additional 70 kcal/kg/day is required. Parenterally fed infants, compared with those enterally fed, begin to grow at a lower energy intake because of smaller faecal energy losses and reduced energy expenditure. Nevertheless, the goal energy intake for a rapidly growing preterm infant is about 120 kcal/kg/day (see Ch. 16 part 1 ), or even higher in long-term ventilated infants with chronic lung disease, whose energy requirements are increased by 25–30% ( ).
Protein
The goal of supplying protein to the neonate is to achieve nitrogen retention at in utero rates without causing metabolic disturbance. Preterm infants will lose approximately 1% of their total protein stores each day if they are given glucose alone ( ). Amino acids therefore need to be started in the first 24 hours of life. A minimum of 1.5 g/kg/day is recommended in order to prevent catabolism, although this is insufficient to support growth ( ; ). Commonly the amino acid intake is then increased slowly over the next few days because of concerns about the ability of the preterm infant to metabolise protein. However a number of investigators have examined higher early amino acid infusion rates, ranging from 2.4 to 3.5 g/kg/day ( ; ; ). All reported improved nitrogen retention without any adverse effects in the short term. It may be that starting amino acids at full rates, or at least increasing rapidly, will go some way to minimising the time to regain birthweight.
Optimal parenteral protein requirements for growth, as determined by a variety of methods, are in the range 3.5–4.0 g/kg/day for extremely low birthweight infants and 3.2–3.8 g/kg/day for very low birthweight infants ( ). The amount of amino acids required to achieve adequate catch-up growth, if there has been a period of poor early weight gain, is likely to be higher than this ( ). The recommended requirements for term infants are somewhat lower at 2.0–3.0 g/kg/day ( ).
Preterm infants require not only more amino acids than term infants but also qualitatively different amino acids. Cysteine, taurine, tyrosine and histidine have been considered as semiessential amino acids in preterm infants ( ). Conversion of methionine to cysteine and taurine, and of phenylalanine to tyrosine, is affected by enzyme immaturity. However, the addition of cysteine ( ) and taurine ( ) to PN solutions in preterm infants did not improve nitrogen retention or weight gain. Taurine is important in the conjugation of bile acids, although humans can also conjugate bile acids with glycine. Some investigators consider that the addition of taurine to total PN (TPN) reduces the incidence of cholestasis ( ). Comparison of amino acid solutions based on the composition of egg protein and breast milk has shown that the latter results in a lower risk of high plasma phenylalanine levels but a higher risk of low tyrosine levels ( ; ). No adverse neurodevelopmental outcome has been observed after hyperphenylalaninaemia induced by PN ( ). If adequate non-protein energy is provided, the risk of hyperphenylalaninaemia is reduced (intravenous energy intake of >34 kcal/g protein). Amino acid solutions designed for paediatric patients have been shown to result in a more favourable plasma aminogram, higher nitrogen retention and better weight gain in preterm infants ( ; ). Current amino acid preparations available for neonates are based on the plasma aminograms of either cord blood or breastfed term infants.
In order to utilise the amino acids efficiently, sufficient non-nitrogen energy must be provided. Energy intake should be at least 40–50 kcal/kg/day for optimal amino acid utilisation, because, with lower energy intakes, more of the infused amino acids are oxidised to meet endogenous energy needs and less remain for tissue synthesis. found that, when energy intakes of more than 70 kcal/kg/day were given to preterm infants, the major determinant of nitrogen retention was the protein intake. Whether this non-protein energy is derived from glucose or fat makes no difference to the nitrogen-sparing effect.
Ammonia, plasma amino acid profiles and urea levels have all been used to monitor the tolerance and adequacy of amino acid intake in the clinical setting. Current neonatal preparations are well tolerated and thus monitoring ammonia and amino acid levels is no longer required routinely. Urea levels are monitored regularly and some clinicians will limit amino acid intakes in the presence of rising urea. However urea levels reflect hydration status, renal function and illness severity and have been shown not to correlate with protein intake ( ). In contrast a low urea may reflect inadequate intake.
Carbohydrate
The goal of carbohydrate provision for the neonate is to maintain euglycaemia and promote optimal growth and body composition.
The consensus interpretation of the neurophysiological and neurodevelopmental outcome data is currently that neonatal blood glucose concentration should be maintained above 2.6 mmol/l ( Ch. 34.1 ). The risks of hyperglycaemia and glycosuria increase with decreasing gestation and birthweight ( ).
The rate of endogenous glucose metabolism in well, fasting neonates has been estimated to be 4–6 mg/kg/min ( ). Some premature infants will require more than this to maintain a satisfactory blood glucose. Glucose infusions should be commenced at 4 mg/kg/min (10% dextrose at 60 ml/kg/day will provide an infusion rate of 4 mg/kg/min) and increased as required. If a concentration of more than 12.5% dextrose is required, this should be given centrally owing to the risks of subcutaneous tissue infiltration. Since glucose tolerance improves with increasing postnatal age the glucose infusion rate can usually be progressively increased. Excessive carbohydrate will theoretically increase CO 2 production, although it is uncertain whether this is ever of clinical relevance. It may also impair liver function by inducing the storage of triglycerides and other fatty acids within the liver cells. The European Society of Paediatric Gastroenterology, Hepatology and Nutrition recommends that preterm infants’ maximum glucose intake should be 8.3 mg/kg/min while term infants may tolerate up to 13 mg/kg/min (18 g/kg/day) ( ).
If hyperglycaemia occurs, the baby should be carefully assessed. Hyperglycaemia occurring in a previously metabolically stable baby may be the first sign of infection, although immature infants may have limited tolerance of intravenous glucose even when infused at physiological rates. Hyperglycaemia during glucose infusion appears to be due primarily to persistent endogenous hepatic glucose production secondary to an insensitivity of hepatocytes to insulin ( ). Insulin can be infused in infants who remain hyperglycaemic at a glucose infusion rate of 8 g/kg/day (6 mg/kg/min) ( ), commencing at 0.05 units/kg/h. A randomised controlled trial in infants of below 1000 g birthweight with glucose intolerance has shown that insulin therapy improves glucose intake and weight gain ( ). However the practice of prescribing early insulin replacement therapy in order to increase the glucose intake in very low birthweight infants appears to offer little clinical benefit and may be associated with increased risk ( ) and is not currently recommended ( ).
Lipid
The goals of providing fat to neonates are to prevent fatty acid deficiency, facilitate provision of lipid soluble vitamins and promote optimal growth and body composition.
Nitrogen sparing effects of carbohydrate and fat are similar in parenterally fed infants, although lipid has the benefit of being calorie-dense ( ; ). Abnormal plasma fatty acid patterns have been noted within 2–3 days of lipid free alimentation, with a deficiency state in 10 days ( ). Essential fatty acid deficiency can be prevented by as little as 0.5 g/kg/day of Intralipid ( ), a fat emulsion derived from soybean oil containing 54% linoleic acid and 8% linolenic acid. While soybean-based emulsions have been the gold standard for many years, the balance of ω-3 and ω-6 fatty acids is probably not ideal for the preterm infant. Linoleic acid (an ω-6 polyunsaturated fatty acid (PUFA)) is the dominant fatty acid in soy-based emulsions. However there is concern that this has the potential to inhibit the production of ω-3 PUFAs, such as docosahexaenoic acid (needed for retinal and brain development). As the metabolites of the ω-3 and ω-6 fatty acids are anti-inflammatory and proinflammatory respectively, an imbalance may have an impact on the infant’s immune response. Olive oil-based emulsions contain oleic acid (ω-9 long-chain monounsaturated) as the main fatty acid and therefore may provide a more appropriate balance between ω-6 and ω-3 fatty acids. However studies have not shown any clinically significant effect to date ( ). Fat emulsions containing equal proportions of long- and medium-chain triglycerides (LCT/MCT) have been used in infants, and one study showed greater nitrogen retention with LCT/MCT than with LCT emulsions ( ).
Phytosterols found in soybean oils are thought to contribute to PN-associated liver disease. This, in addition to the proinflammatory effects of linoleic acid, has led to discontinuation of soy lipid emulsion as the mainstay of treatment. Fish oil has a high concentration of eicosoapentaenoic acid, docosahexaenoic acid and ω-3 fatty acids. Recent experience has suggested that fish oil monotherapy has a potentially beneficial effect in infants with PN-related liver disease ( ). The development of lipid emulsions combining soy, olive and fish oils and MCTs offers a potentially beneficial product which is currently being evaluated in the preterm population.
Lipid utilisation is limited and often unpredictable in preterm and in small-for-gestational-age infants, owing to deficient cellular uptake and utilisation of free fatty acids rather than low lipoprotein lipase activity ( ). Carnitine plays an important role in the oxidation of fatty acids by facilitating their transport across mitochondrial membranes. Preterm infants fed parenterally with carnitine-free solutions develop low blood and tissue carnitine concentrations because of their small carnitine depots and limited capacity for carnitine biosynthesis. Carnitine supplementation, however, does not improve growth or lipid tolerance and is not recommended ( ).
There have been a number of concerns over the early use of lipid; however, randomised controlled trials have established the benefits and safety of parenteral fat commenced on the day of birth ( ; ; ). Concern had been expressed about the effects of lipid infusion on respiratory function. However oxygenation and pulmonary haemodynamics in infants with severe respiratory distress syndrome were not affected when parenteral fat was infused at a dose of 1–4 g/kg/day ( ; ) but deteriorated when the infusion rate exceeded an equivalent of 6–7 g/kg/day ( ). An association between parenteral fat administration and coagulase-negative staphylococcal bacteraemia in infants has been found ( ) but there is no evidence that it impairs immune function in infants ( ; ). An increase in circulating free fatty acid levels can theoretically compete with bilirubin for binding to albumin. However, fat emulsion is also capable of binding unconjugated bilirubin ( ) and infusions of 2–4 g/kg/day have been found to have no effect on total or unbound serum bilirubin ( ). At levels of free fatty acids, bilirubin and albumin usually occurring in this population, significant displacement does not occur ( ).
Free radicals generated when soy lipid emulsion undergoes peroxidation could be potentially damaging. Light-induced formation of triglyceride hydroperoxides can be prevented by covering the lipid emulsion with aluminium foil, although this has largely fallen out of fashion ( ). There is also evidence suggesting that failure to photoprotect PN contributes to high blood glucose and triglyceride levels ( ). Lipid tolerance can be improved by using a continual infusion over 24 hours and by using a 20% rather than 10% concentration. Compared with 10% Intralipid, 20% Intralipid has a lower phospholipid/triglyceride ratio and liposomal content, and thus results in lower plasma triglyceride, cholesterol and phospholipid concentrations ( ).
A 20% lipid emulsion should be started at 1 g/kg/24 h and increased daily by 1 g/kg to 3 g/kg/24 h as tolerated ( ). Plasma triglyceride levels should be monitored (see Table 17.4 , below), as plasma turbidity assessed by visual inspection or nephelometry does not reliably predict serum concentration ( ). If the baby has poor growth but is tolerating lipids, the total dose can be increased to 3.5 g/kg/day. When triglyceride levels exceed 2.0 mmol/l, it is necessary to reduce or interrupt fat infusion until normal values are regained. The lipid infusion should be reduced or interrupted for 24–48 hours during acute sepsis, because of the reduced fat oxidation rate ( ).
Minerals
Early hypernatraemia in preterm infants is caused mainly by their high insensible water loss ( Ch. 18 , Ch. 15 ), while early hyponatraemia is caused mainly by water overload. Late hyponatraemia in preterm infants is due to limited tubular sodium reabsorption, and diuretic therapy may contribute ( ). No sodium should be added to intravenous fluids or PN until postnatal natriuresis has occurred ( Ch. 18 ). Thereafter, 3–5 mmol/kg/day is recommended, to prevent late hyponatraemia, and further increased if the infant is receiving furosemide for chronic lung disease or has significant ongoing renal losses. Although a potassium intake of 1–2 mmol/kg/day is required for the growing preterm infant, it should be withheld in the first 3 days after birth in those who are extremely preterm, because they are at risk of developing non-oliguric hyperkalaemia from immature distal tubular function. Hypochloraemic alkalosis is prevented by a chloride intake of 2 mmol/kg/day. Chloride intakes in excess of 6 mmol/kg/day are inadvisable because of the risk of hyperchloraemic metabolic acidosis ( ). Hyperchloraemic acidosis can be avoided by replacing part of the sodium chloride load with sodium acetate ( ).
Parenteral administration of calcium at 1 mmol/kg/day from birth can reduce early neonatal hypocalcaemia in preterm infants ( ). Requirements calculated to match intrauterine accretion rates in a rapidly growing preterm infant are, however, higher than those used to maintain short-term homeostasis. PN solutions should contain 1.3–1.5 mmol/100 ml of calcium and phosphorus (molar ratio of 1 : 1 or a ratio of 1.3 : 1 by weight) and 0.2–0.3 mmol/100 ml of magnesium. Factors that affect solubility of calcium and phosphorus are discussed below (see Techniques , below). High intakes of calcium and phosphorus should only be given through a central venous line.
Trace elements and vitamins
Table 17.2 summarises the recommendations on parenteral minerals and trace elements for preterm infants based on expert consensus published by ). Trace elements are added routinely to the aqueous component of PN. Zinc and selenium levels should be monitored after the first month of prolonged PN, particularly in infants with underlying GI pathology. Because selenium and chromium are excreted mainly through the kidneys, intake may need to be reduced when renal function is impaired. The aluminium content of PN infusates may be up to 1 µmol/dl as a result of aluminium contamination of the components used, such as calcium gluconate, which can contribute up to 80% of the total aluminium load. As it has been suggested that this will adversely affect neurodevelopment, aluminium contamination should be reduced as far as possible ( ).
Sodium | 3–5 mmol |
Chloride | 3–7 mmol |
Potassium | 2–3 mmol |
Calcium | 1.5–2.0 mmol |
Phosphorus | 1.5–1.9 mmol |
Magnesium | 0.2–0.3 mmol |
Zinc | 6.1 µmol |
Copper | 0.3 µmol |
Selenium | 19–57 nmol |
Manganese | 18.2 nmol |
Iodine | 7.9 nmol |
Chromium | 1–5.8 nmol |
Molybdenum | 2.6 nmol |
Paediatric multivitamin preparations are available ( ). Water-soluble vitamins can be added to either the aqueous or the lipid component. Fat-soluble vitamins should be added to the lipid component. If they are added to the amino acid solution as part of a multivitamin preparation, about 80% of vitamin A and 30% of vitamins D and E is lost during administration owing to adherence to tubing and photodegradation, especially during phototherapy ( ; ). By adding the vitamin preparation into the fat emulsion instead of the amino acid–glucose mixture, vitamin losses can be reduced and the risk of deficiency minimised ( ; ). A dose of 4 ml/kg Vitlipid (Fresenius Kabi) will give an adequate amount of vitamin A ( Table 17.3 ). Fat-soluble vitamin levels should be monitored in all babies requiring more than 1 month’s PN.
RECOMMENDATIONS | COMPOSITION of VITLIPID (per ml) | |
---|---|---|
Vitamin A | 700–1500 IU | 230 units |
Vitamin D | 40–160 IU | 40 units |
Vitamin E | 2.8–3.5 IU | 0.7 units |
Vitamin K | 10 µg | 20 µg |
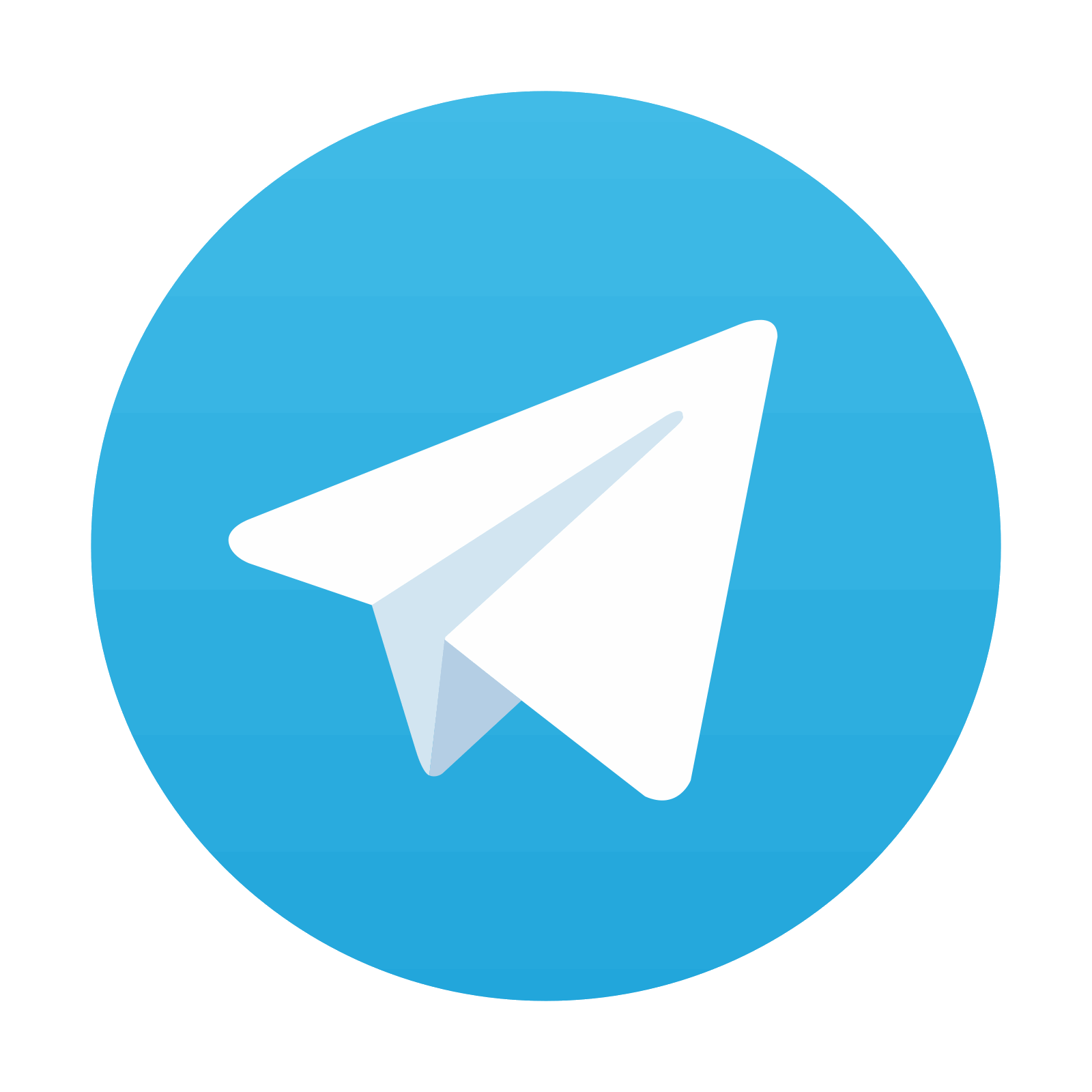
Stay updated, free articles. Join our Telegram channel
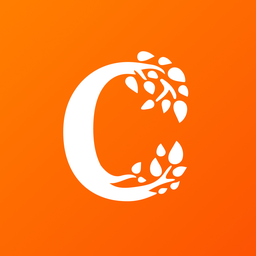
Full access? Get Clinical Tree
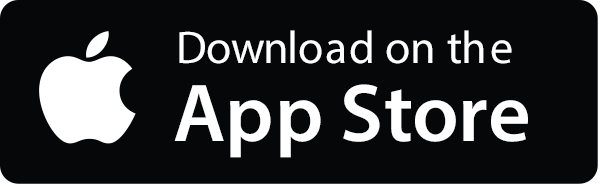
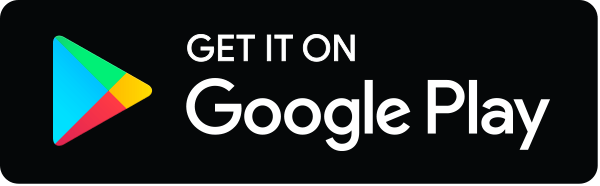