History of the Use of Oxygen in Clinical Medicine
Although anecdotal information indicates that oxygen was already known by the Chinese in the thirteenth century, our present knowledge of its chemical and biological characteristics and its clinical application derives from the discoveries made almost simultaneously by C.W. Scheele (Sweden), Joseph Priestley (Britain), and Antoine-Laurent de Lavoisier (France). By heating mercury oxide, silver carbonate, magnesium nitrate, and potassium nitrate, they all produced the same gas previously known as phlogiston and that later was identified as a source of life because it allowed the survival of a mouse in a sealed jar, while without addition of this gas the mouse would die. Moreover, Priestley showed that plants were capable of producing it, and thus he opened the path for further studies on photosynthesis. The name oxygen comes from the Greek words oxys (acrid) and gene (something that produces something), meaning a substance that produces acids. Still today, in German oxygen is known as Sauerstoff, or acidic substance.
Oxygen was first used in neonatology in the eighteenth century for the resuscitation of newborn infants. At the beginning of the twentieth century, oxygen was infused in the umbilical vessels of asphyxiated infants or directly given into the pharynx or via a gastric catheter. In the 1930s oxygen started to be used liberally in the treatment of preterm infants suffering respiratory distress. With the ongoing use of oxygen, clinical scientists started to describe its positive and negative effects undoubtedly associated with its restricted or liberal use in the treatment of neonatal patients. Oxygen when liberally used was identified as the agent that caused what was initially called retrolental fibroplasia (RLF), characterized by the formation of a thick membrane in the retrolental space that resulted in damage and detachment of the retina and frequently blindness. This severe ophthalmologic condition had already caused blindness in about 10,000 infants in the early 1950s. Randomized trials performed in 1954-1956 clearly showed that the liberal use of oxygen was a major cause of RLF. It is important to note that at that time the level of oxygenation of the patients could be monitored only by taking into account variables such as respiratory rate, heart rate, and/or color. Beginning in the 1960s, objective oxygen monitoring, such as blood gas analysis, transcutaneous oxygen monitoring, and later on pulse oximetry, was incorporated into the routine of care of preterm infants, allowing a more precise means of supervising oxygen status and supplementation.
On the other hand, the establishment of a causative relationship between excessive oxygen and RLF pushed the pendulum to the opposite extreme, and oxygen was drastically limited even in the most severe cases of respiratory distress. Prolonged and extreme hypoxemia resulted in an exponential increase in cerebral palsy and mortality from respiratory failure; thus for every baby saved from RLF, 16 died from respiratory insufficiency.
Since 1995, experimental and clinical research has exponentially increased our knowledge of oxygen metabolism and its toxic consequences in the neonatal period. The front line of neonatal research is directed especially at three different stages during the perinatal period when oxygen is most frequently needed: (1) the fetal-to-neonatal transition and postnatal adaptation—that is, the need for oxygen in the delivery room; (2) the oxygen saturation target ranges when oxygen is supplemented in the neonatal intensive care unit (NICU); and (3) the need for oxygen at home after hospital discharge of patients with chronic conditions.
This chapter is meant to provide a comprehensive approach to the relevant basic, metabolic, and clinical aspects related to oxygen therapy in the newborn period.
Basic Principles of Oxygen Physiology
Aerobic Metabolism
Oxygen (O 2 ) is one of the most abundant elements in nature; the second most abundant component of breathing air, constituting 21% of its composition; and probably the most widely used drug in neonatology. The presence of oxygen will allow the complete combustion of glucose, amino acids, and free fatty acids in a highly efficient process that produces approximately 20 times more energy than anaerobic combustion. This process takes place within the mitochondria, which act as the energy factories of the cell ( Fig. 16-1 ). Substrates are metabolized into acetyl coenzyme A, which enters the tricarboxylic acid cycle (Krebs cycle) where energy in the form of highly energized electrons is liberated and transported by specific proteins (NADH, NADPH, FADH) to the electron transport chain (ETC) located in the inner mitochondrial membrane. Electrons, considered reducing equivalents, provide the energy necessary to maintain the electrochemical gradient that drives adenosine triphosphate (ATP) synthesis. Components of the ETC pump protons across the inner mitochondrial membrane against an electrochemical gradient, and the protons are taken in again by ATP synthase. In this process, energy is recovered and employed to transform adenosine diphosphate into ATP. Electrons are captured by oxygen thus permitting the formation of water and avoiding electron leakage and formation of free radicals; thus, each molecule of dioxygen will be completely reduced by four electrons. Metabolic substrates used to provide energy are highly organ specific; thus, the central nervous system and erythrocytes almost exclusively depend on glucose, whereas cardiac contraction uses energy provided from the combustion mainly of free fatty acids. Oxidative phosphorylation provides most of the ATP needed by the body and is especially relevant in aerobic-dependent tissues known as oxyregulators, such as brain. These organs cannot adapt for even short periods of time in the absence of oxygen without undergoing necrosis and/or apoptosis.

Reactive Oxygen Species, Redox Regulation, and Antioxidant Enzymes
The term reactive oxygen species (ROS) refers to a series of molecules derived from incomplete reduction of molecular dioxygen ( Fig. 16-2 ). Even under physiologic conditions, a small percentage of electrons will “leak,” causing a “partial” reduction of oxygen (oxygen with fewer than four electrons). The incomplete reduction of oxygen with one electron elicits the production of anion superoxide (•O 2 − ), [•O 2 − ] with the addition of two electrons will lead to the generation of hydrogen peroxide (H 2 O 2 ), and with a third electron, the highly reactive hydroxyl radical (OH) will be produced. ROS are also relevant in the regulation of nitric oxide (NO) metabolism and availability and therefore indirectly have an important influence on airway and vascular reactivity. Hence, when superoxide anion binds to NO, peroxynitrite (ONOO − ) will be produced. Peroxynitrite is a highly reactive nitrogen species but also influences vascular reactivity in such areas as the lung, where its production can lead to increased vasoconstriction, causing pulmonary hypertension. The production of ROS under physiologic and also pathologic conditions is highly dependent on the concentration of oxygen in the tissue. ROS are produced in hypoxic and hyperoxic situations and especially when the oxygen concentration fluctuates from hyperoxia to hypoxia and vice versa. ROS can be extremely aggressive when acting as free radicals, causing direct structural and/or functional damage and/or interfering with essential redox-regulatory elements. In other circumstances, ROS can be relatively stable and act as signaling molecules in physiologic processes.

The term free radical refers to any molecule capable of independent existence with one or more unpaired electrons in the outer shell (e.g., anion superoxide, hydroxyl radical). Free radicals form covalent bonds to share one electron with other molecules; however, the resulting molecule easily decomposes, leading to the formation of toxic products. Free radicals may also react with nonradical molecules in typical chain reactions, causing damage to DNA, proteins, and lipids or promoting the formation of adducts with DNA. Under very stressful conditions (ischemia–reperfusion, inflammation, or hyperoxia) damage caused by free radicals can lead to cell death by necrosis or apoptosis or marked cellular dysfunction. In addition to mitochondrial respiration, ROS are produced also by the cytochrome P450 monooxygenase system, xanthine oxidoreductase, nitric oxide synthases, heme oxygenases, and other enzymes involved in inflammatory processes. Moreover, in the presence of transition metals such as iron, copper, zinc, and manganese, the generation of ROS can be exponentially increased.
ROS also have a relevant role in cell physiology. At low/moderate concentrations, especially those ROS that are not free radicals (e.g., hydrogen peroxide) elicit an ample array of cellular responses acting as signaling molecules. Thus, ROS-mediated actions can be protective against ROS-induced oxidative stress and reestablish or maintain redox homeostasis. One of the most important roles of ROS is the regulation of NO production, the oxidative burst produced as a response to infectious agents by phagocytic NAD(P)H oxidase, or acting as sensing elements for regulating tissue oxygen needs, cell adhesion, immune responses, or induced apoptosis.
Redox Regulation
The concept of oxidative stress as a global imbalance affecting the entire economy no longer adequately explains redox biology. Central sulfur–disulfide couples, which include reduced and oxidized glutathione (GSH/GSSG), cysteine/cystine, and reduced and oxidized thioredoxin, function as reducing counterparts of H 2 O 2 and other oxidants in controlling the redox state of oxidizable thiols in proteins. These sulfur switches in which hydrogen peroxide has a relevant role are used for cell signaling, protein structure, protein trafficking, and regulation of enzyme, transporter, receptor, and transcription factor activity. Redox mechanisms control proinflammatory and profibrotic signaling, cell proliferation, apoptosis, and a range of other biologic processes by modifying the protein structure without involving oxidative structural or functional changes. This concept differs from previous thinking in which the importance of free radical mechanisms and macromolecular damage as an underlying mechanism was overemphasized.
Antioxidant Defenses
The redox balance at various sites in our organism requires the intervention of antioxidant defenses to counterbalance the generation of ROS. These mechanisms include both enzymatic and nonenzymatic processes. Antioxidant enzymes, through catalytic reactions, remove ROS and protect proteins through the use of chaperones, transition metal-containing proteins (transferrin, ferritin, ceruloplasmin), and low-molecular-weight compounds that function as oxidizing or reducing agents. Superoxide dismutases (SODs) constitute a family of enzymes located in the cytoplasm (Cu/Zn SOD), in the mitochondria (Mn/Cu SOD), or extracellularly (Zn/Cu SOD), which convert or dismutate superoxide anions to H 2 O 2 . Catalases and glutathione peroxidases (GPx) convert H 2 O 2 into H 2 O and O 2 . GPx couples H 2 O 2 reduction to water with the oxidation of GSH to GSSG. GSSG is again reduced to GSH by the activity of the pentose shunt. Other systems that detoxify hydrogen peroxide in mitochondria and other organelles include glutaredoxin, thioredoxin, thioredoxin reductase, and the peroxiredoxins. Other enzymes with antioxidant and signaling functions are heme oxygenases (HO-1 and HO-2). HO-1 removes heme, a prooxidant, and generates biliverdin, an antioxidant-releasing iron, and carbon monoxide. Finally, nonenzymatic antioxidants such as GSH, vitamin C, vitamin E, and β-carotene also function to protect cells from the damaging effects of ROS. The ontogeny of enzymatic antioxidant expression progresses gradually during gestation. Compared to full-term infants, preterm infants have immature antioxidant defenses, which are more susceptible to ROS-associated conditions derived from hypoxia–reoxygenation, inflammation, or infection. In addition, transplacental passage of antioxidants also occurs late in gestation. In assays performed in human abortus materials, it has been shown that antioxidant enzyme activities in response to oxidant insults increase with advancing gestation. As a consequence, conditions occurring during pregnancy such as preeclampsia significantly alter placental antioxidant enzyme expression, causing a prooxidant burden for the fetus.
Biomarkers of Oxidative Stress
Oxidative stress can be assessed by various means. Direct damage to molecules such as proteins, lipids, and DNA can be measured, determining the results of the interaction with free radicals on their chemical structure. However, oxidative stress can also be indirectly measured by increased activity of antioxidant enzymes or increment in the concentration of oxidized antioxidants such as the GSH/GSSG ratio. In clinical research, determination of the concentration derived from free radical aggregation in biofluids such as blood, plasma, serum, urine, or cerebrospinal fluid has gained popularity. Table 16-1 summarizes the most widely employed biomarkers of oxidative stress. The GSH/GSSG ratio is a comprehensive indicator of redox status and can be determined in whole blood. Lipid peroxidation is determined by measuring malondialdehyde in blood or urine. However, isoprostanes, reflecting non-cyclooxygenase oxidation of arachidonic acid, are now widely employed because they are very stable and not influenced by diet, parenteral nutrition, or gestational age. The preferred method is liquid chromatography coupled to mass spectrometry. Isofurans, which reflect the oxidation of arachidonic acid under hyperoxic conditions, have been very satisfactorily employed in neonatal studies involving the use of oxygen. Other similar biomarkers are neuroprostanes and neurofurans. Neurofurans reflect the oxidation of docosahexaenoic acid specifically present in the brain and therefore may reflect damage caused by oxidative stress to brain tissue. Damage to proteins can be assessed by mass spectrometry determining ortho – or meta -tyrosine, which reflects the oxidation of circulating phenylalanine in a nonphysiologic metabolic pathway. Finally, determining the oxidation of the guanidine bases in urine or plasma assesses DNA damage, reflecting oxidation of the cell nucleus. There are many other biomarkers (RNA, glycoproteins, exhaled compounds, etc.) under evaluation, but these are still applicable only in more basic research.
Biomarker | Target Molecule | Biologic Effect | Biofluid Determination | Analytical Method |
---|---|---|---|---|
Glutathione (GSH/GSSG ratio) | Antioxidants | General redox status | Total blood | LC-MS/MS |
MDA | Lipids | PUFA peroxidation | Plasma | HPLC (UV detection) |
HNE | Lipids | PUFA peroxidation | Plasma | HPLC |
o -Tyrosine ( o -Tyr/Phe ratio) | Proteins | Tyrosine hydroxylation | Urine | LC-MS/MS |
m -Tyrosine ( m -Tyr/Phe ratio) | Proteins | Tyrosine hydroxylation | Urine | LC-MS/MS |
3N2-Tyrosine | Proteins | Tyrosine nitration | Urine | LC-MS/MS |
8OHdG (8OHdG/2dG ratio) | Lipids | AA peroxidation | Urine/plasma | LC-MS/MS |
F2-IsoPs | Lipids | AA peroxidation | Urine/plasma | GC-MS/MS; LC-MS/MS |
D2/F2-IsoPs | Lipids | AA peroxidation | Urine/plasma | GC-MS/MS; LC-MS/MS |
IsoFs | Lipids | AA peroxidation | Urine/plasma | GC-MS/MS; LC-MS/MS |
NeuPs | Lipids | DHA peroxidation | Urine/plasma | GC-MS/MS; LC-MS/MS |
NeuFs | Lipids | DHA peroxidation | Urine/plasma | GC-MS/MS; LC-MS/MS |
Oxygen-Sensing Mechanisms and Physiologic Response
The following is a brief description of oxygen and hypoxia-inducible factor (HIF-1) interaction and erythropoietin (EPO) expression. Oxygen homeostasis is critical for the survival and function of cells and organisms. On one hand, oxygen in excess (hyperoxia) inevitably causes an excessive production of ROS, causing cell damage. On the other hand, the complete absence of oxygen (anoxia) is lethal. Physiologic systems are, therefore, adapted to achieve the proper equilibrium between oxygen demand and availability to ensure correct cellular functioning. A number of redox-sensitive transcription factors such as HIF-1, cAMP response element-binding protein, nuclear factor κB, activator protein 1, and p53 regulate gene expression in response to changes in the concentration of ROS.
Variations in oxygen concentration in the body elicit two types of responses. During acute hypoxia, pulmonary vasoconstriction and carotid body neurosecretion dependent on increased intracellular calcium levels mediated by ROS are essential for survival. If hypoxia is prolonged, activation of the HIF transcription factors will be triggered. The oxygen level in utero at the time of implantation is around 15 to 20 mm Hg and remains at this low level until the end of the first trimester, providing an adequate environment (reductive) for fetal and placental development. Angiogenesis is stimulated by low oxygen concentrations in tissue through transcriptional and posttranscriptional regulation of growth factors such as vascular endothelial growth factor (VEGF), EPO, placental growth factor, and angiopoietins 1 and 2. The master regulator of the cell’s adaptive response to hypoxia is HIF-1, a heterodimeric transcription factor comprising HIF-1α and HIF-1β subunits. HIF-1α protein is continuously synthesized; however, if the O 2 concentration in tissue is normal or high, it will undergo proteasomal degradation. Under conditions below the specific critical oxygen threshold, HIF-1α protein will be stabilized and will bind to the hypoxia-responsive element of DNA and elicit the expression of numerous genes ( Fig. 16-3 ). HIF-1β is constitutively present in the cell nucleus. Activated genes, and especially VEGF and EPO, are meant to enhance O 2 delivery to tissues.

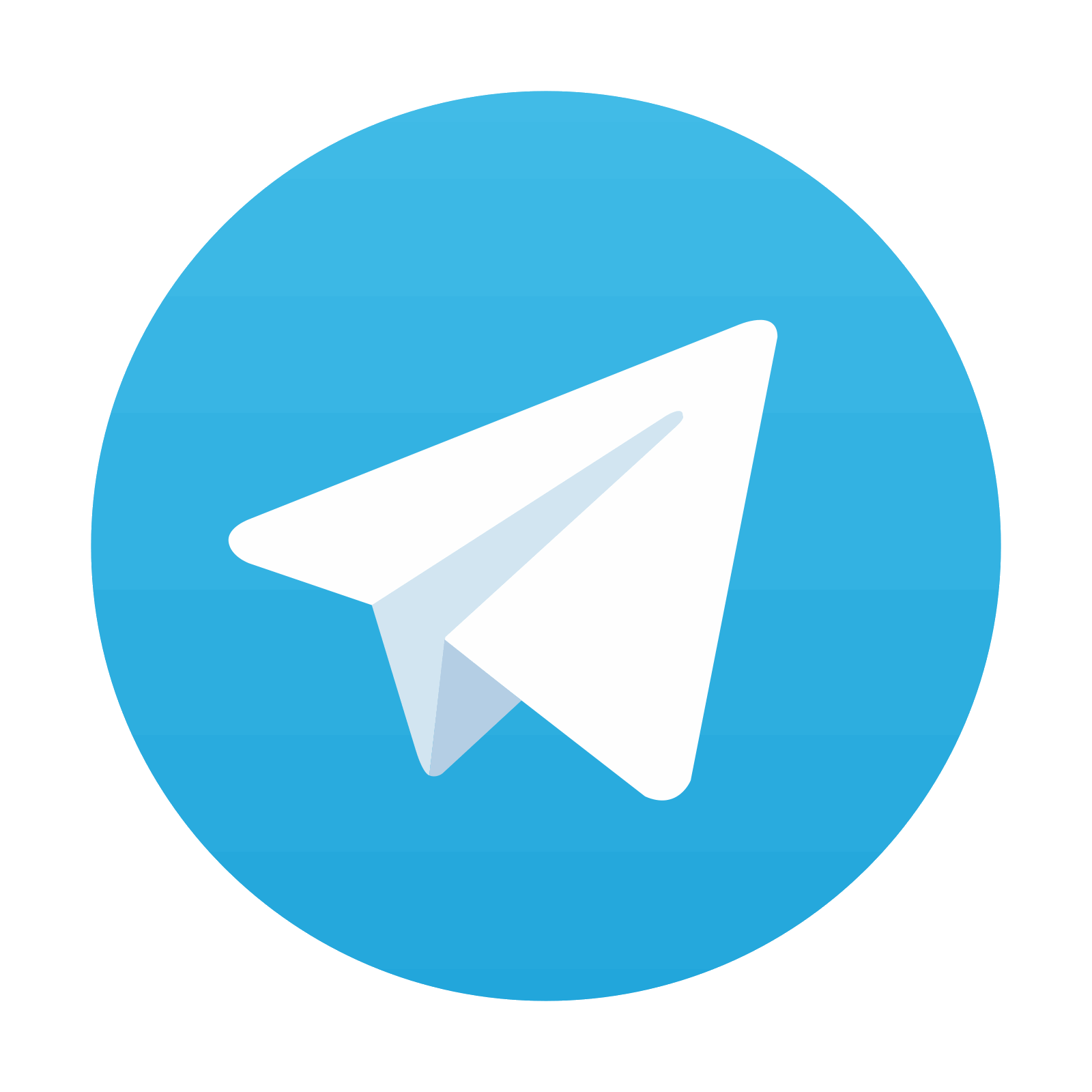
Stay updated, free articles. Join our Telegram channel
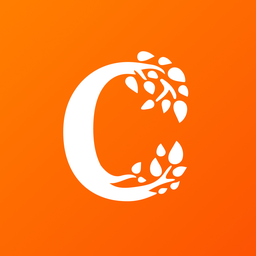
Full access? Get Clinical Tree
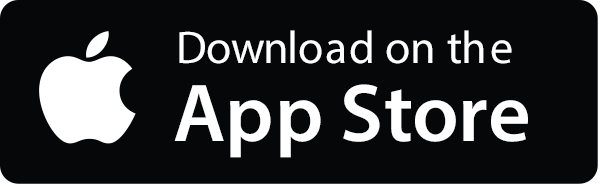
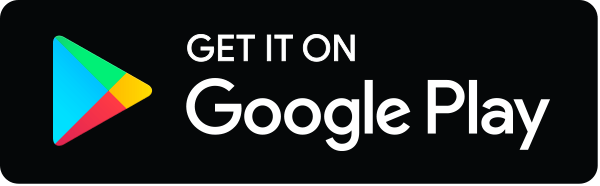