Optimal nutritional support is fundamental for the survival, good health, growth, and development of newborn infants who require intensive care, particularly those who require assisted ventilation. Most of these infants suffer disorders that place them at risk for poor nutrition as a result of increased metabolic rate, limited gastrointestinal function, and limited capacity for metabolic processing of ingested nutrients. Hypoxia, circulatory insufficiency, fluid and electrolyte imbalance, acid–base disorders, and deficiencies in anabolic hormones also complicate the ability to sustain optimal growth. Furthermore, physiologically unstable preterm or “sick” infants often face limitations in the tolerance and processing of enterally and parenterally delivered nutrients. As a result of such poor nutrition, impaired somatic growth is common in these infants, which directly and adversely restricts lung growth and recovery from lung injuries, as well as brain growth, which produces abnormal neurodevelopmental outcomes.
The most widely accepted standard for developing nutritional goals for preterm infants is the intrauterine growth rate of a fetus of corresponding postmenstrual age. Intrauterine growth rates, body composition, and energy expenditure are all used to define the nutritional requirements of the healthy, growing preterm infant. For term infants, the growth and nutrient intake of healthy breast-fed infants are used as references. Surprisingly, much less attention has been paid to how the physiology of illness affects growth, metabolism, and nutrient requirements. For example, the goals of nutritional support for infants who require assisted ventilation are to prevent catabolism and exhaustion of endogenous energy resources, to achieve growth in lean body mass, and to promote healing, growth, and maturation of the lungs, brain, and other vital organs. These nutritional goals must be met without impairing respiratory gas exchange or tissue oxygen delivery. Thus, the nutritional support for infants with respiratory distress syndrome (RDS), chronic lung disease (CLD), and bronchopulmonary dysplasia (BPD) requires special consideration.
Nutritional Requirements
Water Requirement
Water is a key element in nutritional management, because energy, protein, and other nutrients cannot be delivered without water, and optimal water intake is important for all infants who require assisted ventilation. Water is required for growth and is produced in small amounts as a by-product of cellular metabolism. Water comprises a large fraction of body weight in preterm and term infants, declining after birth with improvement in renal function triggered, at least in part, by atrial natriuretic peptide. Body water balance and thus weight may not decrease, however, and in fact often increase with aggressive fluid intake from intravenous (IV) solutions (both dextrose only and total parenteral nutrition (TPN) solutions). Normal infants with good kidney and lung function generally tolerate high rates of IV fluid. In contrast, physiologically unstable infants, such as preterm infants with RDS requiring supplemental oxygen and ventilators, often have poor renal function in the first days after birth. Excessive water intake with limited renal function leads to fluid accumulation in the lung, which worsens lung function and increases the need for potentially injurious oxygen and ventilator support. Knowledge of the factors that affect water balance is essential in estimating water requirements, especially for preterm and critically ill infants who require assisted ventilation, because excessive water balance, particularly with increased salt intake, can compromise lung function. Pulmonary edema due to fluid overload can contribute to decreased pulmonary compliance and increased airway resistance. A Cochrane review showed that restricted water intake significantly reduced the risk of patent ductus arteriosus and tended to reduce the risk of BPD.
Insensible Water Loss
Smaller, less mature infants lose more water by evaporation because of greater surface area–to–body weight ratios, thinner and more permeable skin, dry ambient air, higher respiratory rates, and increased humidity gradient between the upper respiratory airway and the gas mixture they breathe. Antenatal corticosteroids given to promote fetal maturation reduce the insensible water loss (IWL) of very preterm infants during the first few days of life, presumably by enhancing epidermal barrier function. Several devices also have been shown to decrease IWL. Transparent thermal blankets can reduce IWL by preterm infants in incubators. Polyethylene occlusive skin wrapping also reduces the IWL of infants under radiant warmers, including during resuscitation in the delivery room. In recent years, IWL has been much reduced with improved humidification of incubators, ventilators, and respiratory gas circuits that allow spontaneous breathing by the infant, such as continuous positive airway pressure (CPAP) or heated high-flow nasal cannula.
Renal Water Excretion
The amount of water required for urine production depends on the renal solute load and the renal concentrating ability. The term newborn infant can produce urine as dilute as 50 mOsm per liter and can concentrate to a maximum osmolality only slightly above plasma. The preterm infant’s kidney is limited to a narrower range of concentrating ability, producing a higher renal sodium excretion rate.
The renal solute load comes from two sources: exogenous , primarily electrolytes and metabolic products of nutrients, and endogenous , products of catabolism or changes in body composition. In practice, the amount of solute excreted by the kidney is larger than the exogenous or potential solute load, because many of these infants are in a catabolic state; in such cases, the exogenous solute intake is usually low, so that the actual solute excretion is still generally less than what the sick or physiologically unstable infant’s kidneys can excrete (about 35 mOsm/kg/day). In contrast, growing infants deposit some of the exogenous solute in the body (approximately 1 mOsm per gram of weight gain) so that the actual solute load is less than the potential. An infant receiving preterm formula with a potential renal solute load of 24 mOsm/100 kcal at 125 kcal/kg/day (30 mOsm/kg/day) and gaining 15 g/kg/day of weight would excrete about 15 mOsm/kg/day. Avoiding excess solute load is desirable, particularly to avoid accumulation of salts and water in the lung that could lead to increased oxygen and ventilator requirements. It is unusual, though, with modern TPN solutions, enteral nutrient mixtures, and enteral supplements, to exceed renal solute excretion capacity, even in very preterm infants.
The critically ill infant who requires assisted ventilation may have special problems with renal handling of water. Hypoxia or hypotension during the course of the illness may compromise the kidney function by causing tubular or cortical injury. During the anuric or oliguric phase of acute tubular necrosis (ATN), the renal water excretion is markedly reduced, and its allowance in the maintenance water calculation should be reduced accordingly. In the diuretic phase of ATN, water excretion through the kidney is markedly increased.
The renal function of infants with RDS is similar to that of infants of the same gestational age without respiratory disease so long as normal blood gas and acid–base status can be maintained. However, in a large case series, RDS was an independent predictor of renal impairment, presumably due to associated hypoxia and acidosis that may have reduced renal perfusion. Most preterm infants, including those with RDS, have an increase in urine volume on the second or third day of life, which is accompanied by a rise in plasma atrial natriuretic peptide and contraction of the extracellular water compartment. These changes are often temporally associated with improvement in respiratory function. There is no evidence, however, that renal function is affected by specific methods of assisted ventilation, such as conventional ventilation or high-frequency oscillation. Furthermore, there is no evidence to support routine administration of diuretic therapy to promote diuresis in preterm infants with RDS.
Energy Requirement
Healthy preterm infants gain weight at rates similar to the fetus of corresponding postmenstrual age (15 to 18 g/kg/day). An average intake of 110 to 130 kcal/kg/day from preterm infant formula or human milk is generally sufficient to support normal growth and metabolism of very low birth-weight preterm infants, provided protein intake is adequate. This broad range of energy intake is designed to accommodate the needs of rapidly growing, extremely low birth-weight infants, who may need higher energy delivery for growth, as well as larger, more mature preterm infants who may require less. Moreover, infants receiving high energy intakes develop excess body fat without accelerating their gain in fat-free mass. The energy from the diet that is not lost in the stool or urine (about 10% of intake) is either expended for metabolism or stored in the body for growth. Energy is expended during rest (about 50 kcal/kg/day for preterm infants) and also for activity, thermoregulation, postprandial or synthetic energy expenditure, and growth ( Table 29-1 ). Calorie needs vary depending on whether the infant is enterally or parenterally fed. The energy expended during growth in human infants has been estimated to be 5.5 kcal/g of new tissue deposited for protein and 1.6 kcal/g for fat. Estimates of the overall energy cost of growth range from about 2.9 to 6.0 kcal/g. The energy cost of physical activity in healthy, preterm infants accounts for only about 3% of the total daily energy expenditure.
Nutrient | ADVISABLE INTAKE (PER KG/DAY) | |
---|---|---|
<1000-g Infant | 1000- to 1500-g Infant | |
Energy (kcal) | 110-130 | 110 |
Protein (g) | 3.5-4.5 | 3.5-4.0 |
Sodium (mmol) | 3.6 | 3.0 |
Potassium (mmol) | 2.5 | 2.2 |
Chloride (mmol) | 3.2 | 2.6 |
Calcium (mg) | 150-220 | 120-200 |
Phosphorus (mg) | 60-140 | 60-140 |
Magnesium (mg) | 8-15 | 8-15 |
Iron (mg) | 3.0-4.0 | 2.0-3.0 |
Zinc (mg) | 1.0-3.0 | 1.0-3.0 |
Copper (mg) | 0.2 | 0.2 |
Energy demands, oxygen consumption, and resting energy expenditure are 15% to 25% higher in infants with respiratory disease such as apnea, RDS, and BPD and those on mechanical ventilation, an important factor to consider when providing nutrition to this vulnerable population. Energy expenditure is increased during therapy with caffeine for apnea of prematurity. Preterm infants with RDS have increased oxygen consumption in the first 1 to 2 days of life, and oxygen consumption is directly correlated with the amount of ventilator support the infant requires.
Energy expenditure is increased with more severe RDS requiring higher levels of ventilatory support and oxygen administration, which together are associated with higher risk of BPD. Spontaneously breathing infants with BPD also have increased rates of energy expenditure, in conjunction with lower energy intake and poor weight gain. Increased oxygen consumption is not entirely due to increased work of breathing, however, as strategies implemented to improve pulmonary function do not necessarily decrease oxygen consumption. Low energy intake (<100 kcal/kg/day) also increases the risk of severe retinopathy of prematurity (ROP) in extremely preterm infants.
Protein Requirement
The provision of parenteral or enteral protein soon after birth is essential to prevent negative nitrogen balance and promote protein accretion. Preterm infants have lower lean mass and higher body fat by postmenstrual age 40 weeks compared to normally growing fetuses at term. Specifically, infants with BPD have decreased lean body mass, indicating that current levels of protein intake might be inadequate. Thus, providing an optimal balance of protein and energy is critically important to maximize lean tissue and organ growth. The protein requirement of the healthy term infant in the first month of life is approximately 2.0 g/kg/day. Estimates of the protein requirement of preterm infants are higher than for term infants and range between 3.5 and 4.5 g/kg/day depending on gestational age (see Table 29-1 ). These estimates are supported by experimental studies of protein turnover, nitrogen balance, tissue accretion, and growth.
Preterm infants, including those who require assisted ventilation, benefit from IV amino acids starting as soon after birth as possible. In a retrospective analysis of a large multicenter database, early initiation of IV amino acids was associated with better growth, as assessed by gain in weight, length, and head circumference from birth to 36 weeks’ postmenstrual age. Although this study did not evaluate lung growth specifically, linear and head growth measurements are better indicators of lean tissue growth (including the lung) than weight measurements alone. Furthermore, the severity of BPD is reduced among infants who receive more nutrition during the first 3 weeks after birth. In adults, branched-chain amino acids such as leucine in IV nutrient solutions can modulate respiratory distress, decreasing P co 2 and stimulating the ventilatory response to hypercarbia, corresponding to an enhanced ventilatory sensitivity. In preterm infants of ∼30 weeks’ gestation, providing higher concentrations of branched-chain amino acids in parenteral nutrition results in increased dynamic lung compliance, decreased pulmonary resistance, and fewer episodes of apnea, findings also observed in neonatal piglet studies. Finally, infants given a nutrient-enriched formula through 3 months of age demonstrate greater linear growth and lean and bone mass growth when fed higher intakes of protein, calcium, phosphorus, and zinc than are provided in standard formulas.
Lipid Requirement
Lipids are important as a source of energy for metabolism and growth. For preterm infants, a reasonable range of fat intake is 4.4 to 6.0 g of fat per 100 kcal (40% to 55% of energy intake). Only two fatty acids are known to be essential (cannot be synthesized in the body) for humans: α-linolenic acid, an ω3 fatty acid, and linoleic acid, an ω6 fatty acid. Milk and most formulas contain adequate amounts of these two essential fatty acids, linoleic acid and α-linolenic acid, from which the essential long-chain polyunsaturated fatty acids (LC-PUFAs), such as arachidonic acid (AA) and docosahexaenoic acid (DHA), respectively, are derived. These LC-PUFAs play particularly important biological roles in development as immune modulators and components of membrane phospholipids and are essential for normal development of the central nervous system. Preterm infants have insufficient capacity for de novo synthesis of LC-PUFAs, requiring that these substrates are received from the diet. For infants fed intravenously, the requirement for LC-PUFAs can be met by providing 0.5 to 1.0 g/kg/day intake in the form of IV lipid emulsion.
Lipid emulsions are a key component of parenteral feeding regimens for infants who require assisted ventilation, providing an important source of energy. Early introduction of soybean oil–based emulsions in preterm infants was shown to have detrimental effects on pulmonary gas exchange and hemodynamics, though a meta-analysis of this practice did not show beneficial or adverse effects on growth, death, or CLD.
ω3 LC-PUFAs such as DHA and AA are found in human milk. There is no evidence from clinical trials that adding LC-PUFAs to commercial term infant formulas confers benefits. However, based on theoretical benefits that they could improve visual acuity, neurodevelopmental outcomes, and lung development, and evidence from studies that show no harm, they are commonly added to commercial infant formulas.
Decreased DHA and AA levels in preterm infants are associated with an increased risk of BPD. Preterm infants born to mothers randomized to high-dose supplements to augment DHA in their breast milk showed a reduced incidence of BPD compared to standard-dose supplements. It might be that LC-PUFA requirements for extremely low birth-weight infants are much higher than what is seen in term breast milk; thus, higher supplemental doses of DHA might be of greater benefit and require further investigation.
Carbohydrate Requirement
Approximately half of an infant’s energy needs are normally provided by carbohydrate metabolism. Moreover, glucose is the primary energy source for brain metabolism. In the preterm infant, glucose is largely derived from exogenous carbohydrate sources once glycogenolysis has exhausted stored hepatic glucose. Gluconeogenesis develops soon after birth and is not easily suppressed by increases in plasma glucose or insulin concentrations, thus contributing to a common problem of hyperglycemia in preterm infants. Maintenance of normal plasma glucose concentrations is fundamental, as the vital organs (brain and heart) take up glucose according to plasma concentration and not IV infusion rates or rates of hepatic glucose production. When plasma glucose concentrations decline, the newborn brain may use ketone bodies as additional energy sources, but these are usually limited in very preterm and small-for-gestational-age infants with intrauterine growth restriction (IUGR) who have low body stores of fat.
Preterm and stressed infants are at risk for developing hyperglycemia, especially those with respiratory distress, mechanical ventilation, and restricted cardiac output and circulation. Such infants often are intermittently hypoxic, which adds to increased glucagon, adrenaline, and glucocorticoid secretion. These stress-reactive hormones reduce insulin secretion and insulin action and promote glucose production from both glycogenolysis (acutely) and gluconeogenesis (over a sustained period). They also contribute to protein breakdown. Hyperglycemia leads to increased cellular allostatic load, in which excess carbon cannot be fully oxidized, producing increased amounts of reactive oxygen species that cause cellular breakdown ( Table 29-2 ).
Excess Glucose, Cortisol, and Mitochondrial Allostatic Load (Acute as well as Chronic) |
Increased cortisol and catecholamine levels |
Hyperglycemia |
Increased insulin ∗ |
Increased cell glucose uptake |
Increased Mitochondrial Allostatic Load |
Mitochondrial fragmentation |
Increased reactive oxygen species production |
Accumulation of mitochondrial DNA damage |
Decreased energy-producing capacity |
Increased susceptibility to cell death |
Cellular Dysfunction |
Oxidative stress |
Molecular damage |
Telomere shortening |
Cell loss, apoptosis |
Energy deficiency |
Systemic Inflammation |
Increased circulating proinflammatory cytokines |
∗ Also antiinflammatory, by suppressing proinflammatory transcription factors (nuclear factor B, activator protein-1, and early growth response-1).
Other complications of hyperglycemia frequently develop in neonates when maximal glucose oxidative capacity (>12 mg/kg/min) is exceeded, including increased energy expenditure (glucose-to-fat synthesis is energy expensive), increased oxygen consumption (and hypoxia), increased carbon dioxide production, increased fat deposition in excess of lean mass, and increased fatty infiltration of heart and liver. Such problems may underlie the increased morbidity and mortality in infants with severe and/or persistent hyperglycemia.
Mechanisms for enteral carbohydrate digestion and absorption mature in a defined sequence in the human fetus. Sucrase, maltase, and isomaltase are usually fully active by 24 to 28 weeks’ gestation, but lactase lags behind the others and is not fully active until term. For this reason, most preterm formulas contain lower lactose content than that in human milk. However, there is evidence that intestinal lactase activity increases to adequate functional levels within a few days after the initiation of enteral feedings, even in infants born as early as 28 weeks’ gestation. Furthermore, despite the late gestational rise in lactase activity, both term and preterm infants seem to tolerate the carbohydrates in human milk and commercial formulas quite well. Activity of pancreatic amylase remains low until after term. Salivary amylase activity is present even in very preterm infants.
Other carbohydrates such as mannose and inositol, as well as oligosaccharides (prebiotics), play an important role in nutrition and organ development for the preterm infant. Mannose is an essential carbohydrate for protein glycosylation and normal neural development. Mannose is a component of oligosaccharides, which contribute to the establishment of nonpathogenic intestinal flora, and play a major role in intestinal health for both term and preterm infants. Inositol is particularly important for lung development, as it is integral in the formation of surfactant phospholipid production. Inositol is present in high concentrations in human milk and can be synthesized by newborn infants of gestational age 33 weeks or more. There is evidence that lower inositol concentrations are associated with more severe RDS and that inositol supplementation may reduce the incidence of RDS. Benefits of routinely supplementing preterm infant nutrition with inositol are being investigated.
Mineral Requirements
The requirements of sodium, potassium, and chloride are all between 2 and 4 mmol/kg/day (see Table 29-1 ). The requirement of sodium may be even higher for preterm infants, particularly in the first week of life because of urinary sodium losses. Urinary excretion of electrolytes depends on intake. Typical urinary concentrations of sodium are 20 to 40 mmol/L and of potassium 10 to 30 mmol/L. When the infant is receiving diuretic therapy, urinary sodium concentrations can reach 70 mmol/L or higher, and these large losses must be considered when electrolyte needs are assessed. This is particularly important as insufficient sodium intake impairs longitudinal growth and weight gain.
The recommended intakes of calcium and phosphorus depend on the route of administration. If given enterally, absorption rates might be limiting. The fraction of calcium absorbed depends on the type of milk or formula and the infant’s gestational and postnatal age. If given parenterally, the limits of mineral solubility become a significant factor. Adequate enteral intake of calcium for term infants in the first 6 months of life is about 70 mg/kg/day. The calcium requirement for preterm infants is much higher, 150 to 220 mg/kg/day, because of more active bone formation and remodeling (see Table 29-1 ). Adequate enteral intake of phosphorus for term infants is ∼30 mg/kg/day but is higher for preterm infants (60 to 140 mg/kg/day) to ensure adequate bone mineralization. Fortification of human milk is necessary to ensure adequate intakes of calcium and phosphorus to preterm infants who are fed human milk by bottle or tube, particularly with mature mother’s milk and donor milk. Recommended IV intakes of calcium and phosphorus are lower than enteral requirements, ranging from 65 to 100 mg/kg/day for calcium and 50 to 80 mg/kg/day for phosphorus. The risk factors in preterm infants for calcium and phosphorus deficiency and subsequent rickets are gestational age less than 27 weeks or birth weight less than 1000 g, long-term parenteral nutrition (>4 to 5 weeks), severe BPD requiring diuretics and fluid restriction, long-term steroid treatment, a history of necrotizing enterocolitis (NEC), and intolerance to enteral formula or human milk.
Adequate intake of magnesium for enterally fed term and preterm infants is about 10 mg/kg/day (see Table 29-1 ). Intravenous intake of 7 to 10 mg/kg/day is recommended for infants receiving TPN for prolonged periods.
Iron intake should be 2 to 4 mg/kg/day for both term and preterm infants who are enterally fed but increased to 6 mg/kg/day for preterm infants with anemia of prematurity who are receiving recombinant erythropoietin. Up to three-fourths of preterm infants will develop iron deficiency anemia in their first half-year of life if they do not receive enough iron from supplements or formula. One study found improved neurocognitive outcomes when iron supplements were started at 2 weeks vs 8 weeks after birth, and a recent meta-analysis found improved mental performance and psychomotor development when iron supplements were started soon after birth. The American Academy of Pediatrics guidelines recommend that 2 to 4 mg/kg/day of iron should be provided to growing preterm infants beginning at 2 weeks of age. Risk of iron overload and toxicity (e.g., possible increased risk of ROP, increased risk of infection, and impaired growth) from unnecessary iron supplementation following red blood cell transfusions also must be considered ; commonly, iron supplementation is delayed for 2 weeks after a transfusion. Each red blood cell transfusion in preterm infants typically adds 8 mg/kg iron, and hepatic iron stores as well as serum ferritin concentrations are highly correlated to the number of blood transfusions received. Formula-fed infants should receive iron-fortified formula, beginning with the first formula feedings, to help counter anemia of prematurity and to ensure adequate iron supply to the growing brain, as iron is preferentially taken up by red blood cells. Because individual infants vary considerably in their hematocrit at birth, delayed cord blood clamping, transfusions, blood sampling, and erythropoietin treatment, it is helpful to monitor their iron status and need for iron supplementation by following serum ferritin concentrations. Research to define the benefits and risks of iron supplementation and fortification of milk and formula in preterm infants is sorely lacking.
The advisable enteral intakes of zinc and copper are 1 to 3 and 0.1 to 0.2 mg/kg/day, respectively. Zinc has been shown to improve growth specifically in extremely low birth-weight preterm infants with CLD receiving human milk. Other minerals such as selenium, manganese, iodine, chromium, and molybdenum are also required in trace amounts ( Table 29-3 ).
Advisable Intake (per day) | |
---|---|
Vitamin A | 400-3330 IU/kg |
Vitamin D | 400-1000 IU |
Vitamin E | 3.3-16.4 IU/kg |
Vitamin K | 4.4-28 μg/kg |
Vitamin C | 20-55 mg/kg |
Thiamin (B1) | 140-300 μg/kg |
Riboflavin (B2) | 200-400 μg/kg |
Niacin (B3) | 1.0-5.5 mg/kg |
Pyridoxine (B6) | 50-300 μg/kg |
Biotin | 1.7-16.5 μg/kg |
Pantothenic acid | 0.5-2.1 mg |
Folic acid | 35-100 μg |
Cobalamin (B12) | 0.1-0.8 μg/kg |
Vitamin Requirements
Vitamin A is essential for growth and differentiation of epithelial tissues, including those in the lung. Preterm infants have low stores of vitamin A at birth, and preterm infants with lung disease have lower plasma vitamin A levels than those without lung disease. Thus, vitamin A deficiency may contribute to the development of BPD. A large randomized clinical trial showed a reduction in the risk of death or BPD (defined as a requirement for supplemental oxygen at 36 weeks’ postmenstrual age) with vitamin A supplementation, 5000 IU given intramuscularly three times a week for 4 weeks. A systematic review of eight clinical trials of vitamin A supplementation to preterm infants confirmed the beneficial effect of vitamin A in reducing the risk of death or oxygen requirement at 1 month of age by 7% (relative risk 0.93, 95% confidence interval 0.88 to 0.99). Neurodevelopmental assessment of surviving infants showed no differences between groups at 18 to 22 months’ corrected age. The number needed to treat was 20 to allow survival of one more infant without BPD. In the review, other morbidities and mortality were not significantly reduced by vitamin A supplementation. Despite its efficacy, vitamin A supplementation has not become standard practice, in part because of the need for repeated intramuscular injections, as absorption from the gastrointestinal tract does not appear to be sufficient.
Vitamin D is essential for bone health. As with other fat-soluble vitamins, the body stores of vitamin D are low at birth, especially in preterm infants. Infants who require assisted ventilation have no exposure to ultraviolet light in the hospital and limited exposure after discharge and thus have minimal cutaneous synthesis of vitamin D. The recommended enteral intake of vitamin D is 200 to 400 IU per day regardless of body weight. This dose is probably sufficient to maintain adequate (>50 nmol/L) serum concentrations of vitamin D and to prevent vitamin D-deficiency–associated rickets. Higher doses (800 to 1000 IU per day) have been recommended for vitamin D–deficient newborns (see Table 29-3 ). The major circulating metabolite of vitamin D after its 25-hydroxylation in the liver is 25-hydroxyvitamin D. Levels may be monitored in infants at risk for developing rickets, including those infants with extreme prematurity, short bowel syndrome, cholestasis, and long-term exposure to diuretics and/or steroids.
Vitamin E is a major natural antioxidant in the body. It protects lipid-containing cell membranes against oxidative injury and is thought to play a role in preventing neonatal oxygen toxicity. The recommended enteral vitamin E intake, 3.3 to 16.4 IU/kg/day, is sufficient to compensate for variation in vitamin E absorption and distribution and in the intake of other nutrients known to influence vitamin E requirement, such as iron and LC-PUFAs. The amount of vitamin E required to prevent lipid peroxidation in vulnerable tissues depends on the PUFA content of the tissues and diet. Thus, it also is advisable to keep the dietary ratio of vitamin E to PUFAs at or above the level of 0.6 mg of d -α-tocopherol (0.9 IU) per gram of PUFAs. Recommended IV doses of vitamin E are 2.8 IU/kg/day as α-tocopheryl acetate. Though studies have shown efficacy of vitamin E supplementation in reducing the risk of ROP and intraventricular hemorrhage, excessive intake of vitamin E has the potential for severe toxicity including risk of sepsis. Consequently, routine administration of high-dose vitamin E supplements is not recommended, even though a single enteral dose of vitamin E at birth may help correct the relative vitamin E deficiency that has been found in some very preterm infants in the first days of life.
Vitamin K is essential to prevent hemorrhagic disease of the newborn in the first weeks of life. A single dose of intramuscular vitamin K (1.0 mg) is effective in the prevention of classic hemorrhagic disease of the newborn. Subsequent supplementation with vitamin K is necessary to prevent deficiency, especially for critically ill infants, who often receive broad-spectrum antibiotics (reducing vitamin K synthesis by gut bacteria) and may have other abnormalities of hemostasis or hepatic function.
Infants with fat malabsorption due to cholestatic liver disease or short bowel syndrome are at risk for developing fat-soluble vitamin deficiency. Thus, additional fat-soluble vitamin supplementation may be necessary in these patients. Additional nutrients, such as water-soluble vitamins and other trace substances, are required for recovery and healthy growth of preterm infants (see Table 29-3 ).
Parenteral Nutrition
Parenteral nutritional support is essential for providing a continuous supply of both macro- and micronutrients to the preterm infant starting immediately after birth and as enteral feedings are being advanced, particularly in those infants who cannot tolerate enteral feedings.
Intravenous Access
Indwelling short polyvinyl chloride catheters provide safe and convenient access to the peripheral venous circulation. Hand and arm sites are preferred, but scalp veins are sometimes necessary. The most common significant complication of peripheral IV infusions is tissue necrosis at the site of extravasation. The infusion site must be carefully observed to detect extravasation as soon as possible. Infusions with glucose concentrations greater than 12.5 g/dL should be avoided in peripheral veins.
Central vein catheters, usually Silastic, are used for longer term IV delivery of nutrients to newborn infants. Umbilical vein catheters are placed immediately after birth into most preterm and sick term infants who require iv nutrition and other treatments. The percutaneously inserted central catheter is the preferred technique for long-term central venous access. With good care, the risk of infectious complications is very low, but removing such lines as soon as possible is essential to reduce rates of line sepsis and other complications such as thrombosis or extravasation of infused fluid into the pleural or pericardial space. The surgically placed central vein catheter is typically used for infants who have required gastrointestinal surgery for gut malformation (especially gastroschisis) or NEC and for whom extensively prolonged parenteral nutrition is anticipated.
Composition of Total Parenteral Nutrition
Preterm infants who are ill enough to require assisted ventilation should receive IV nutrition as soon as possible after birth. The initial infusion should consist of 10% dextrose in water to prevent hypoglycemia, though occasionally this concentration of dextrose will induce hyperglycemia in the extremely low birth-weight infant. It is now abundantly clear that providing IV amino acids to sick preterm infants as soon as possible after birth can improve protein balance and can increase protein accretion. Most neonatal intensive care units now use a “starter” TPN solution that provides at least 2 g/kg/day of amino acids in addition to 10% dextrose. A relative restriction of water intake during the first day or two of life allows for a physiologic state of negative water and sodium balance that accompanies the mobilization of extracellular water. A standard starting rate for IV fluids is 80 mL/kg/day, with titration of the infusion rate based on environmental humidity (more for infants under open warmers, less for infants in high-humidity incubators), use of humidified air–oxygen mixes in ventilator or CPAP circuits, and when the onset of diuresis and natriuresis develops.
Sodium is added by day 3 to deliver 2.5 to 3.6 mmol/kg/day, provided the serum sodium concentration is not elevated. Initially withholding sodium from preterm infants who have RDS in the first 24 to 48 hours of life helps reduce water accumulation in the lung. The sodium is usually given as sodium chloride or sodium acetate, depending on the degree of metabolic acidosis. Potassium chloride or acetate also is added to the infusate on day 2 or 3 to give 2.0 to 2.5 mmol/kg/day, provided the serum potassium concentration is normal and adequate urine flow is established. By the end of the first week of life, maintenance delivery of sodium and potassium should be achieved (see Table 29-1 ).
Intravenous calcium and phosphorus supplementation should be started in high-risk infants as soon as possible after birth, if the serum calcium level is low, and certainly if the infant is showing signs of hypocalcemia (tremulousness, seizures, apnea, or cardiac arrhythmia). The usual dosing range of parenteral calcium in the form of calcium gluconate is 0.6 to 2.5 mmol/kg/day (25 to 100 mg/kg/day of elemental calcium), and the dose is titrated based on either the total serum calcium level (with consideration for albumin binding) or the ionized calcium level (with consideration for cardiac output and neurologic status). Phosphorus is given as sodium phosphate with a dosing range of 0.75 to 2.5 mmol/kg/day (18 to 80 mg/kg/day of phosphate). The Ca/P ratio should be kept at 1:1 on a molar basis and 1.3:1 on a mg/mg basis to maximize accretion of both minerals. Caution should be used if calcium is infused into a peripheral IV catheter, as tissue necrosis and sloughing can occur. Some intensive care units avoid peripheral calcium administration entirely except in emergencies (hypocalcemic seizures, shock). Magnesium supplementation ranges from 0.12 to 0.4 mmol/kg/day (3 to 10 mg/kg/day).
Full IV amino acid nutrition, including carbohydrate, protein, minerals, and vitamins, should be started as soon as possible after birth, preferably within the first 24 hours, and should be continued at this rate if a delay in advancement to full enteral feedings is anticipated. Lipid emulsions should normally be given as a part of any infant’s parenteral nutrition regimen. The IV lipid product in the United States is derived from soybean oil; while it does contain the essential fatty acids, linoleic acid and α-linolenic acid, it lacks their downstream products, AA, eicosapentaenoic acid (EPA), and DHA. This product also contains relatively large amounts of phytosterols, which appear to induce hepatic inflammation and lead to cholestasis and parenteral nutrition-associated liver disease (PNALD). Lipid emulsions must be started within the first few days of life at a minimum dose of 0.5 to 1.0 g/kg/day to avoid essential fatty acid deficiency in infants who cannot be fed enterally. The maximum infusion rate should not exceed 2 to 3 g/kg/day, and the infusion is usually administered over 20 or more hours each day. Hypertriglyceridemia and/or hyperglycemia can result from parenteral lipid administration. Plasma triglyceride levels can be monitored periodically, especially in extremely low birth-weight infants, to assess lipid tolerance. Additionally, IV lipid intake may be associated with decreased binding affinity of bilirubin for plasma protein and increased free bilirubin concentration in very preterm infants.
Newer generation lipid emulsions that contain mainly ω3 LC-PUFAs, such as EPA and DHA, or a mixed emulsion of both ω3 and ω6 LC-PUFAs but with a preponderance of ω3 LC-PUFAs, are available outside of the United States, as of this writing. Evidence from research and practice in other countries demonstrates that the dose and composition of ω3 fatty acids in lipid emulsions may play important roles in reducing development and progression of PNALD.
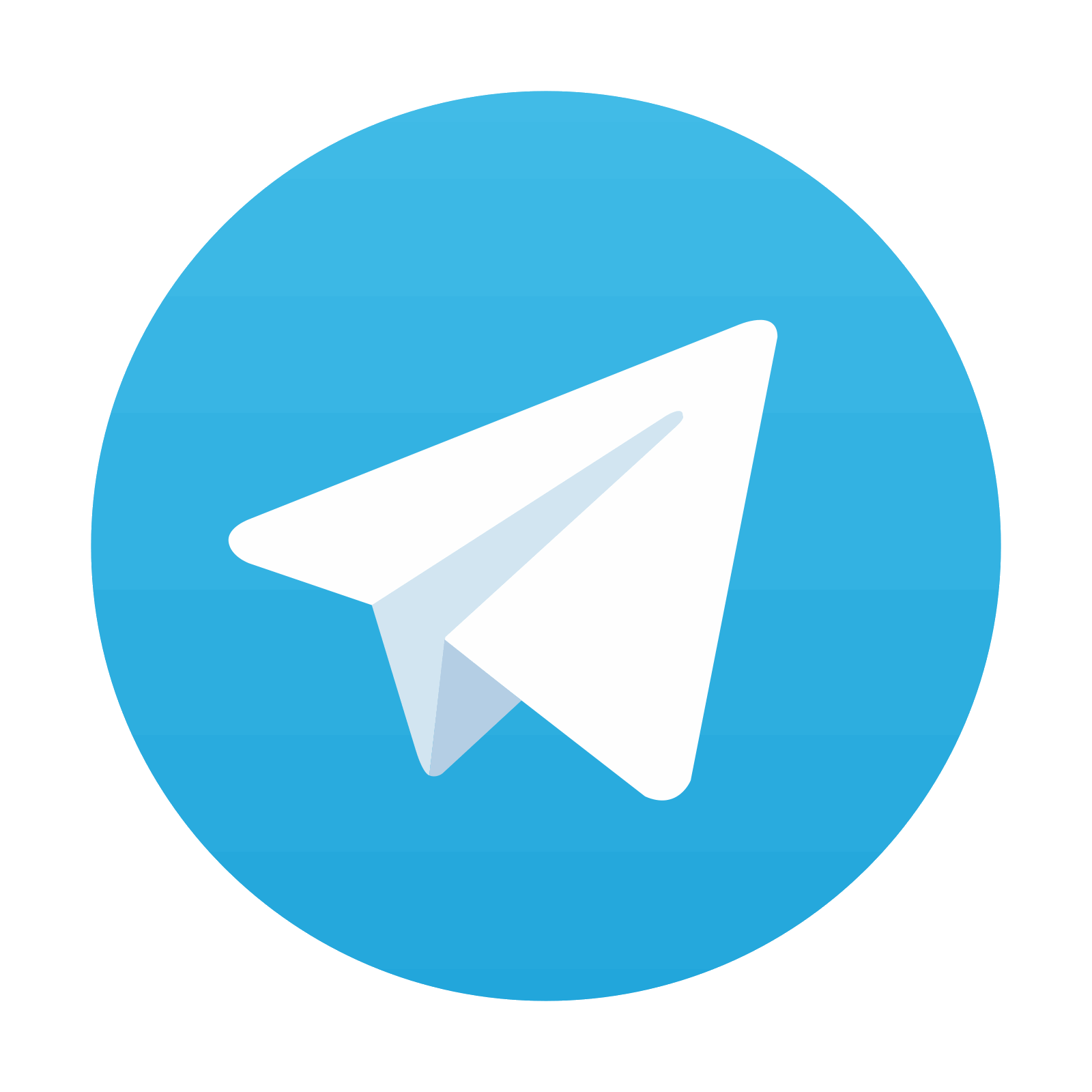
Stay updated, free articles. Join our Telegram channel
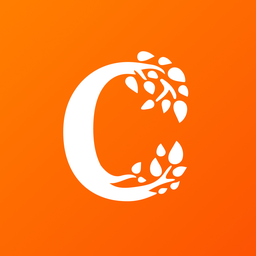
Full access? Get Clinical Tree
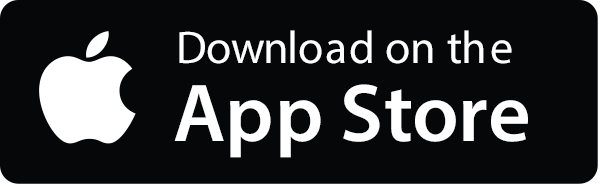
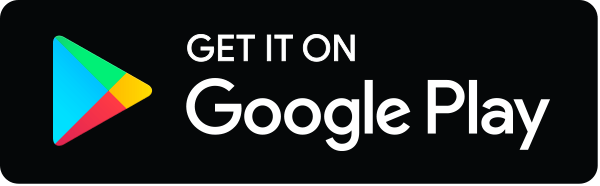