Normal and Abnormal Brain Development
Mechanisms of Brain Development
Normal development of the human central nervous system (CNS) encompasses several steps, including neuroectoderm induction, neurulation, cell proliferation and migration, programmed cell death, neurogenesis and elimination of excess neurons, synaptogenesis, stabilization and elimination of synapses, gliogenesis, and myelination (Table 58-1).
TABLE 58-1
Schematic Chronology of the Major Events During Human Neocortical Development
Neuroectoderm induction | 3rd GW |
Neurulation | 3rd to end of 4th GW |
Prosencephalic and hemispheric formation | 5th-10th GW |
Neuronal proliferation | 10th-20th GW (? 10th-end of gestation for interneurons) |
Neuronal migration | 12th-24th GW (? 10th-41st GW for interneurons) |
Programmed neuronal cell death | 28th-41st GW |
Synaptogenesis | 20th GW to puberty |
Gliogenesis | 20th-24th GW to ? postnatal years |
Myelination | 36th-38th GW to 2-3 postnatal years |
Angiogenesis | 5th-10th GW to ? postnatal years |
These different steps of brain development and maturation are controlled by the interaction between genes and the environment. Numerous genes involved in brain development have been identified: genes controlling neurulation, neuronal proliferation, neuronal size and shape, programmed cell death, neuronal-glial interactions, and synaptic stabilization.1 However, it seems unlikely that the 30,000 genes in humans can totally control the organization of 100 billion neurons and trillions of synapses. A normal pattern of expression of these genes requires an adequate environment. Interactions with the intrauterine milieu (factors coming from the mother, placenta, or amniotic fluid) and with the postnatal environment critically modulate gene expression through reciprocal action with neurotransmitters, trophic factors, and hormones and their machinery. Accordingly, brain malformations can be due to environmental factors, genetic factors, or an interaction of both (Boxes 58-1 to 58-4).
This chapter focuses primarily on the development of the neocortex. Detailed descriptions of the development of other CNS structures such as cerebellum can be found in texts by ten Donkelaar and colleagues2 and Wang and Zoghbi.3
Neural Induction and Neurulation
During early stages of gastrulation, organizing centers produce inductive molecules that initiate genetic programs leading to the differentiation of the neural tissues from surrounding tissues.4 Grafting experiments in amphibians have shown that the appearance of neural tissue depends on signals coming from mesodermic cells of the dorsal marginal zone (the Speeman organizing center) and that other signals deriving from this zone also allow the regionalization of the neuroectoderm along the rostrocaudal axis. Studies have identified some of the factors implicated in neural induction, including follistatin, noggin, Notch, dorsalin1, Wnt1, and Hedgehog.
The anterior neuropore closes at approximately 24 gestational days and the posterior neuropore closes at approximately 26 gestational days. This posterior site of closure is located around the lumbosacral level, and more caudal spinal cord is formed secondarily by a separate process involving canalization (4-7 gestational weeks) and retrogressive differentiation (seventh gestational week to after birth; giving rise to the ventriculus terminalis and the filum terminale). The neural tube is initially a straight structure. Before the closure of the posterior neuropore, the anterior part of the neural tube is shaped into three primary vesicles: the prosencephalon, the mesencephalon, and the rhombencephalon. The prosencephalic phase and the formation of the hemisphere take place between 5 and 10 gestational weeks in humans.
Central nervous system regionalization results from the combination of two mechanisms. Rostrocaudal regionalization creates transverse domains with distinct competences in the neural plate and tube,5–7 whereas dorsoventral regionalization creates longitudinally aligned domains.8 The combination of these two axes yields a grid-shaped pattern or regionalization.9 Different genes involved in this regionalization have been identified. These include BMP (bone morphogenetic proteins), PAX (paired box genes), and SHH (sonic hedgehog) genes for the dorsoventral axis, and HOX (clustered homeobox-containing genes), KROX20, FGF8 (fibroblast growth factor-8), EN (engrailed genes), WNT, OTX (homeobox genes homologous of the Drosophila orthodenticle gene), EMX (related to the “empty spiracles” gene expressed in the developing Drosophila head), and DLX (homeobox genes homologous to the distal-less genes of Drosophila) genes for the rostrocaudal axis (Figure 58-1).

Neuronal Proliferation
There are no precise data concerning the number of neurons present in the brains of different mammalian species. In the human adult brain, estimates range from 3 billion to 100 billion neurons. Similarly the precise proportion of glial cells is unknown, with a neuron-to-glia ratio estimated at between 1 : 1 and 1 : 10.
In some regions of the CNS, neuron production continues for the entire life span. This late neurogenesis is apparent in the olfactory bulb and the dentate gyrus. Its importance at the level of the neocortex, especially in physiologic conditions, remains to be demonstrated. In this context, production of neurons for the human neocortex is generally considered to be a phenomenon occurring during the first half of gestation.
The neocortex is composed of vertical units (neuronal columns): The number of neurons in a given unit seems stable throughout studied mammals and is constant throughout the different cortical areas (with the exception of the visual cortex). In contrast, the time necessary to produce the neocortical neurons of a given column progressively increases with increasing mammalian evolutionary complexity. Indeed, the period of neurogenesis neurons takes 6 days in mice, whereas it takes approximately 10 weeks in humans. Glutamatergic neurons of the neocortex are generated in the ventricular (VZ) and subventricular (SVZ) zones of the lateral ventricles, whereas a large proportion of GABAergic neurons of the neocortex are generated in the ganglionic eminence (which also produces thalamic neurons).
The cerebral cortex has evolved in size by expansion of the surface area, without a comparable increase in its thickness, which imposed folding constraints and convolutions. Recent comparative studies performed during the development of the neocortex in lissencephalic and gyrencephalic models support that increased capacity of progenitors to proliferate, with subsequent amplification of cortical neuron populations, is largely involved in this evolution.
In mice, two main types of progenitors, radial glial cells (RGCs) and intermediate progenitor cells (IPCs), also called basal progenitors, have been described (Figure 58-2).10,11 Radial glial cells occupy the VZ and are characterized by the expression of Pax6. They are highly polarized cells, with their apical pole connected to the ventricle luminal border and their basal pole to the pial surface. They thus extend their cellular process through the entire cortical wall and play a major role in guiding young neurons’ migration into a specific layer. Radial glial cells have the ability to self-renew either by autoreplicative symmetric divisions or by asymmetric division, which generates a further RGC and either an IPC or a neuron (neurogenic division).

Autoreplicative symmetric divisions are prominent in the mouse VZ until embryonic day 13, when the production of neurons becomes amplified through asymmetric neurogenic divisions. Notch activity is required to maintain the basal process and the RGC identity, but the pathways promoting asymmetric versus symmetric divisions remain to be determined. All RGCs give rise to IPCs, which settle in the SVZ. Intermediate progenitor cells express Tbr2, divide symmetrically to self-renew or to give birth to two neurons, and are not polarized. They are limited to one or two further rounds of division in rodents.
In humans, the situation is much more complex (see Figure 58-2).10,11 Whereas RGCs remain the major cell type in the VZ, the SVZ is drastically enlarged and subdivided into the inner (ISVZ) and outer (OSVZ) parts (see Figure 58-2). The ISVZ is mainly composed of IPCs, whereas OSVZ includes IPCs and another type of RGCs, which are not attached at their apical side to the ventricle, but maintain the basal process connected to the pial neocortical surface. These SVZ basal RGCs (bRGCs) express Pax6, similarly to ventricular apical RGCs (aRGCs). Like their apical counterparts, bRGCs can undergo symmetric division to self-renew, and asymmetric divisions to self-renew and generate either IPCs or neurons. Remarkably, both IPCs and bRGCs are endowed with a high ability to proliferate. Their pools are thus largely amplified in humans between the 11th and 17th gestational week, as well as in other gyrencephalic species. Intermediate progenitor cells and bRGCs amplification is obviously an important process that strongly impacts the extent of neurogenesis and appears to be a major process for cortical expansion.
Neuronal Migration and Cortical Lamination
Neocortical neurons derive from the primitive neuroepithelium and migrate to their appropriate position in the cerebral mantle. In humans, migration of neocortical neurons occurs mostly between the 12th and the 24th weeks of gestation.12 The first postmitotic neurons produced in the VZ migrate to form a subpial preplate or primitive plexiform zone (Figure 58-3). Subsequently produced neurons, which will form the cortical plate, migrate into the preplate and split it into the superficial molecular layer (layer I or marginal zone containing Cajal-Retzius neurons) and the deep subplate. Schematically, the successive waves of migratory neurons pass the subplate neurons and end their migratory pathway below layer I, forming successively (but with substantial overlap) cortical layers VI, V, IV, III, and II (an inside-out pattern).

Neocortical migrating neurons can adopt different types of trajectories (Figure 58-4):13,14

1. A large proportion of neurons migrate radially, along radial glial guides, from the germinative zone to the cortical plate. Radial glia are specialized glial cells present in the neocortex during neuronal migration; these cells display a radial shape with a nucleus located in the germinative zone, a basal process attached on the ventricular surface, and a radial apical process reaching the pial surface (Figure 58-5). Rakic15 postulated that these radially arranged glial guides keep a topographic correspondence between a hypothesized protomap present in the germinative zone (ventricular and subventricular zones) and the cortical areas.

2. An important group of neuronal precursors initially adopt a tangential trajectory at the level of the ventricular or subventricular germinative zones before adopting a classic radial migrating pathway along radial glia. This tangential migration could permit some dispersion at the level of the cortical plate of neurons originating from a single clone in the germinative neuroepithelium, increasing the clonal heterogeneity within a given cortical area.
3. Tangentially migrating neurons have also been described at the level of the intermediate zone (prospective white matter). Most of these neuronal cells displaying a migrating pathway orthogonal to radial glia originate in the ganglia eminence. Most GABA-expressing interneurons seem to be produced by this mechanism.
Studies have identified several molecules involved in the control of neuronal migration and in targeting neurons to specific brain regions.16,17 These molecules can be divided into four categories (Figure 58-6):

1. Molecules of the cytoskeleton that play an important role in the initiation and progression (extension of the leading process and nucleokinesis) of neuronal movement. Initiation controlling molecules include Filamin-A (an actin-binding protein involved in periventricular nodular heterotopia) and Arfgef2 (ADP-ribosylation factor GEF2, which plays a role in vesicle trafficking and is involved in periventricular heterotopia combined with microcephaly). Progression controlling molecules include doublecortin (Dcx, a microtubule-associated protein [MAP] involved in double cortex and lissencephaly), Lis1 (a MAP and dynein regulator involved in isolated type 1 lissencephaly and Miller-Dieker syndrome), alpha-tubulin (involved in the formation of tubulin heterodimers), and other molecules that are associated with migration defects in transgenic mice but have not yet been associated with human disorders (phosphatase inhibitor 14-3-3epsilon, MAP1B, MAP2, and Tau).
2. Signaling molecules, which play a role in lamination. These molecules include the glycoprotein Reelin (involved in lissencephaly and cerebellar hypoplasia in humans and in the Reeler mouse mutant characterized by an inverted cortex) and other proteins generally associated with inverted cortex in transgenic or mutant mice, but which have not yet been associated with human disorders such as adaptor protein Disabled-1 (Dab1), ApoE receptor 2 (Apoer2), very low density lipoprotein receptor (Vldlr), two Reelin receptors, serine-threonine kinase Cdk5 (cyclin-dependent kinase 5), activator of Cdk5 p35, Brn1/Brn2, and transcriptional activators of Cdk5 and Dab-1.
3. Molecules modulating glycosylation, which seem to provide stop signals for migrating neurons. These molecules include POMT1 (protein O-mannosyltransferase associated with Walker-Warburg syndrome), POMGnT1 (protein O-mannose beta-1,2-N-acetylglucosaminyltransferase involved in muscle-eye-brain disease), Fukutin (a putative glycosyltransferase involved in Fukuyama muscular dystrophy), and focal-adhesion kinase (Fak involved in migration disorder in transgenic mice). These three human diseases comprise type 2 lissencephaly (cobblestone lissencephaly).
4. In addition to these three major groups of molecules, other factors have been shown to modulate neuronal migration, including neurotransmitters (glutamate and GABA),18–20 trophic factors (brain-derived neurotrophic factor BDNF and thyroid hormones),21 molecules deriving from peroxisomal metabolism, and environmental factors (ethanol and cocaine).
The application of diffusion-weighted magnetic resonance imaging (MRI) or diffusion tensor MRI to the evaluation of developing brain has opened up the possibility of studying some of these developmental events in vivo. Quantitative indices derived from the diffusion tensor allow measurement of apparent diffusion and diffusion anisotropy, which ultimately depend on microstructural tissue development. Several different diffusion tensor imaging (DTI) parameters are available in this assessment. These include the three diffusion tensor Eigen values (11, 12, 13), which represent diffusion along the three tensor principal axes); the mean diffusivity (Dav) or apparent diffusion constant (ADC); and a mathematical measure of anisotropy, which describes the degree to which water diffusion is restricted in one direction relative to all others. Diffusion tensor MRI was used to study cortical development in human infants ranging from 26 to 41 weeks’ gestational age; apparent diffusion of water in cortex was maximally anisotropic at 26 weeks’ gestational age and declined to zero by 36 weeks’ gestational age.22 During this period, the major eigenvector of the diffusion tensor in cerebral cortex is oriented radially across the cortical plate. Vector maps illustrate diffusion anisotropy and direction of the major diffusion eigenvector. In the case of cortical gray matter, the vectors are oriented radially, consistent with the orientation of the radial glial fibers (Figure 58-7).22 Anisotropy changes are different in early intracortical maturation, where changes in fraction anisotropy (FA) are mainly owing to changes in 1123 confirmed also by studies in the developing rat brain.24,25 One study on human fetal brain has shown that cortical anisotropy increases from 15 weeks’ gestation to approximately 26 weeks’ gestation and then shows a gradual decline to 32 weeks’ gestation.26 The increase of anisotropy in this time period coincides with active neuronal migration along the radial glial scaffolding, whereas the decrease coincides with the phase of neocortical maturation with transformation of the radial glia into the more complex astrocytic neuropil.

The use of new, ultrafast MRI sequences such as multiplanar, single-shot, fast spin-echo T2-weighted images provides high-resolution images of the fetus in utero with imaging times of less than 1 minute.27 The normal MRI pattern of fetal brain maturation from 13 weeks’ gestation has been described, documenting the presence of the primary sulci and the insula by 15 weeks’ gestation. Operculization of the insula begins by 20 weeks’ gestation, and all the main sulci, except the occipital sulci, are present by 28 weeks’ gestation.27 From 28 weeks’ gestation there is mainly an increase in secondary and tertiary sulcal formation. From 23 to 28 weeks in vivo28 and from 15 weeks in vitro,29,30 the typical multilayer pattern of the cerebral parenchyma can be observed with the innermost hyperintense signal (T1-weighted MRI) of the germinal matrix (Figure 58-8). The five-layer pattern of the fetal forebrain, including the layers of neuroblast formation and migration, could be identified at 16 to 18 weeks’ gestation in postmortem fetal brains.30 During the last trimester, the gyri and sulci already formed become more prominent and more deeply infolded, with subsequent development of secondary and tertiary gyri at 40 to 44 weeks of gestational age (Figure 58-9).

Central Nervous System Organization
Subplate Neurons
As mentioned, subplate neurons constitute a distinct structure during neocortical development.31 Neurons of the subplate are generated at approximately the seventh week of gestation, and the subplate is generated from the preplate at approximately 10 gestational weeks. This structure localized beneath the neocortical plate reaches its maximal thickness between 22 and 36 gestational weeks. The subplate is present in preterm neonates but has disappeared in full-term neonates. Some authors have suggested that these neurons disappear by apoptosis, whereas others have suggested that they are incorporated into layer VI of the mature neocortex.
The subplate neurons can be lesioned or destroyed in preterm neonates with periventricular white matter lesions.32 These data have been recently substantiated in animal models of periventricular white matter damage (see Chapter 59).33
Magnetic resonance imaging has been used to visualize the developmental evolution of the subplate zone and other laminar compartments of the fetal cerebral wall between 15 and 36 weeks’ gestation. The combination of MRI and histochemical staining of the extracellular matrix enabled selective visualization of the subplate zone in the developing human brain.34 The tissue elements of the subplate zone are embedded in an abundant, very hydrophilic, and transient extracellular matrix that is most likely responsible for the low signal intensity on T1-weighted MRI in Figure 58-10 and the slightly higher signal intensity on T2-weighted MRI as seen in vivo at 26 weeks (Figure 58-11).

Axonal and Dendritic Growth
When neurons near their final destination, they start to produce axons and dendrites, allowing connection with distant cerebral structures. This ontogenic step occurs largely, but not exclusively, during the second half of gestation and extends into the postnatal period. For example, evoked visual potentials can be produced as early as 24 to 27 gestational weeks in human neonates, confirming the existence of an established wiring at this early developmental stage.
Growing axons have to find their path through developing structures to reach their target. In this process, growing axons, and in particular their distal tip, called the growth cone, are helped by several mechanisms:
1. The transcriptome of each neuron contains information determining the types of connections this neuron can establish.
2. Target neurons or neurons on the pathway of growing axons secrete or express on their membranes chemoattraction or chemorepulsion factors that interact with receptors present on growth cones, resulting in attraction or repulsion of these axonal growth cones. Several ligand-receptor families have been described, including netrin, slit, comm, robo, semaphorin, cadherin, and ephrin receptors.35 The interaction among these different ligands and receptors leads to changes in calcium levels in the growth cones, which seem to play a strategic role in the resulting behavior of the growth cones.
3. Neurotransmitters and trophic factors are liberated that will favor or inhibit the extension of the growing axons expressing the corresponding receptor.
4. Growth cones can interact with various glycoproteins of the extracellular matrix that act as guiding cues.
5. At early stages of brain development, distances separating structures are rather small, facilitating the navigation of growth cones. These first-produced axons, called pioneer axons, serve as guides for later-produced axons, when distances between structures are significantly larger.
As for neuronal production, some axonal projections are produced in excess, connecting too many structures or neurons. This initial phase is followed by a regressive phase in which redundant or misconnected axons are eliminated or retracted, allowing the emergence of adequate and functional connections. This balance between the maintenance and the elimination of axons is regulated by different mechanisms. Obviously the survival of the neuron is determinant in this decision. Furthermore, competition for available trophic factors interacts with the genome to modulate this balance.36 Also electrical activity is a strategic determinant for the maintenance of axons. Accordingly, in utero and especially postnatal stimuli and experiences significantly shape the developing brain by modulating the maintenance or elimination of some axons.37
Some callosal connections, some “feedback” intracortical connections, and some corticofugal projections (such as the motor pathway) seem to be examples of connections largely under the control of this “overproduction-elimination” principle and, therefore, are highly dependent on the environment.38 In contrast, some “feedforward” intracortical connections seem to be more genetically predetermined and, therefore, less susceptible to environmental cues.39
Studying white matter development with in vivo imaging was largely impossible before the advent of advanced MR techniques, with diffusion imaging being of particular interest in the assessment of the white matter microstructure. Diffusion characteristics differ between pediatric and adult human brain in two primary ways. First, ADC values are higher for pediatric brain than adult. Second, ADC maps of pediatric brain show contrast between white and gray matter, with the ADC values for white matter being higher than those for gray. The precise cause of the decrease in ADC with increasing age is not known, although it has been postulated that the rapid decrease observed between early gestation and term is a result of the concomitant decrease in overall water content. Brain water content decreases dramatically with increasing gestational age. As it does, structures that hinder water motion (e.g., cell and axonal membranes) become more densely packed, which further restricts the motion of the remaining water. The use of diffusion tensor MRI has allowed visualization of early white matter connectivity (Figure 58-12) with demonstration of interhemispheric callosal fibers in the nonmyelinated stage at 28 weeks of gestation. During white matter development, decreases in diffusion are observed principally in 12 and 13 (and much less in 11), which reflect changes in water diffusion perpendicular to white matter fibers and may indicate changes owing to premyelination (change of axonal width) and myelination.40–42

Relative anisotropy values for white matter areas are relatively low in newborns and increase steadily with increasing age.43 They are particularly low for preterm infants.44 This increase has been attributed to changes in white matter microstructure that accompany the “premyelinating state.” 45 This state is characterized by a number of histologic changes, including an increase in the number of microtubule-associated proteins in axons, an axon caliber change, and a significant increase in the number of oligodendrocytes. It is also associated with changes in the axonal membrane, such as an increase in conduction velocity and changes in Na+/K+–adenosine triphosphatase activity. Myelination then further increases relative anisotropy values. Although myelin accounts for much of the change in relative anisotropy, diffusion anisotropy is present under any circumstance in which the cytoarchitecture of the tissue is arranged in such a way as to lead to greater hindrance of water motion in one direction compared with others.
Making proper connections through white matter structures is probably one of the determining factors for further cortical organization. One major hypothesis for the morphogenetic mechanism of cortical folding is based on mechanical tension along axons in the white matter.46 A striking increase in cerebral cortical volume accompanies the axonal and dendritic growth described previously. That this growth is particularly rapid between approximately 28 and 40 weeks’ gestational age has been shown by quantitative three-dimensional MRI techniques with use of post-acquisition image analysis. Volumetric analysis of MRI datasets is achieved by segmentation of the imaged volume into tissue types depending on their difference in signal intensity, followed by three-dimensional renderings.47 Overall brain volume more than doubles between 28 and 40 weeks’ gestation, and cortical gray matter volume increases fourfold in the same period.48 This increase is thought to relate primarily to neuronal differentiation rather than to an increase in the total number of neurons. Cortical surface, changing from smooth, lissencephalic to highly convoluted, increases fivefold between 28 and 40 weeks’ gestation (Figure 58-13).49

So far, several hypotheses have been put forward on the mechanisms that underlie the folding process during development, but the potential influence of genetic, epigenetic, and environmental factors is still poorly understood. According to postmortem observations of fetal brains,50 the primary folds would form in a relatively stable spatiotemporal way during intrauterine life depending on physical constraints and mechanical factors.51 An attractive theory suggests that the specific location and shape of sulci are determined by the global minimization over the brain of the viscoelastic tensions from white matter fibers connecting cortical areas.52,53 This may explain why specific abnormalities in the sulcal pattern are observed in certain brain developmental disorders that are the result of subtle impairments in the neuronal migration and the setup of corticocortical connections.54,55 The emergence of the cortical foldings in the preterm newborn brain was recently studied by applying dedicated post-processing tools to high-quality MR images acquired shortly after birth over a developmental period critical for the human cortex development.49,56 Through the three-dimensional reconstruction of the developing inner cortical surface, a sulcation index was derived and allowed measurement of variations with age, gender, and presence of brain lesions and mapped the individual sulci appearance, highlighting early interhemispherical structural asymmetries that may be related to the cortical functional specialization of the brain. Females have lower cortical surface and smaller volumes of cortex and white matter than males, but equivalent sulcation. The highest sulcal index is found in the central region, followed by the temporoparieto-occipital region, with the lowest sulcation index in the frontal region, which confirms that the medial surface folds before the lateral surface and that the morphologic differentiation of sulci begins in the central region and progresses in an occipitorostral direction. Such spatiotemporal differences in brain maturation have been described in detail in older children through the analysis of cortical volume and thickness changes.57 In particular, occipital regions grow much faster than prefrontal regions in newborns born at term,58 and higher-order association cortices mature only after lower-order somatosensory and heterotopia visual cortices.59
The right hemisphere presents gyral complexity earlier than the left, which is particularly evident at the level of the superior temporal sulcus, which parallels early functional competence in response to auditive stimuli in newborns.60 Preterm birth may be responsible for the delay that we observed in sulci appearance in comparison with postmortem and fetal studies, in that both cortical volume61 and surface area62 of extremely preterm infants imaged at term equivalent age are decreased and less complex than in normal infants, and this impairment seems to increase with decreasing gestational age at birth.63 Furthermore, preterm infants with intrauterine growth restriction had more pronounced reduction of volume in relation to surface area and increased sulcation with resultant changes in cortical thickness, which correlated with impaired behavioral functions.64
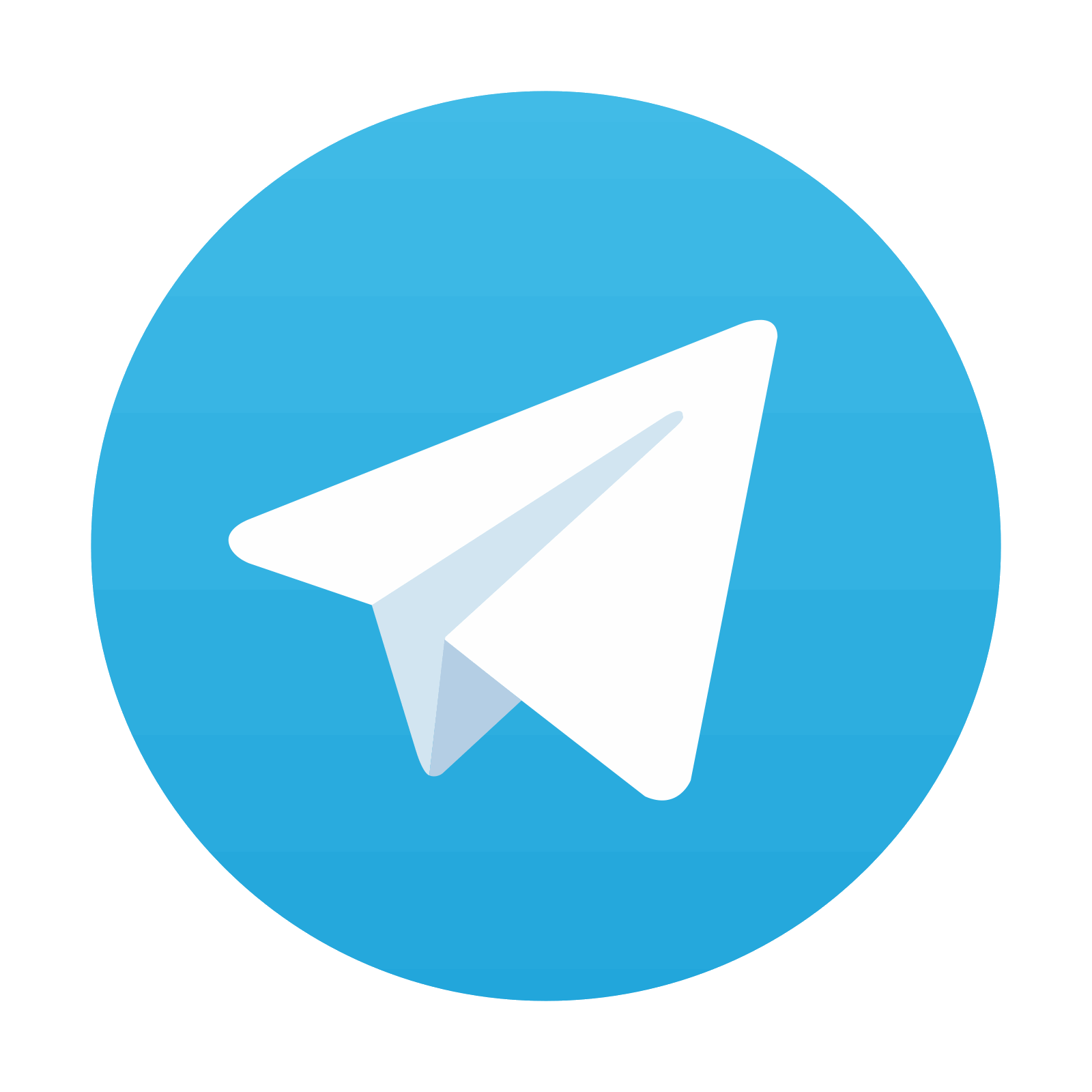
Stay updated, free articles. Join our Telegram channel
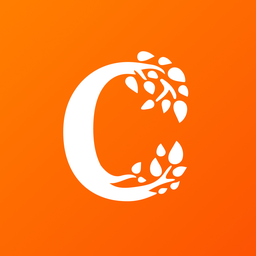
Full access? Get Clinical Tree
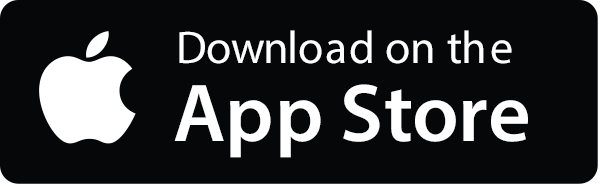
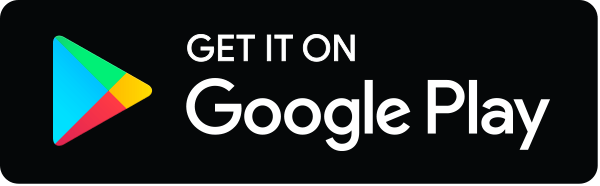