Chapter 17
Nonobstetric Surgery During Pregnancy
Marc Van de Velde MD, PhD
Chapter Outline
MATERNAL SAFETY: ALTERED MATERNAL PHYSIOLOGY
Respiratory System and Acid-Base Balance Changes
Changes in Blood Volume and Blood Constituents
Gastrointestinal System Changes
Altered Responses to Anesthesia
Maternal Cardiac Arrest and Resuscitation
Fetal Monitoring during Surgery
Estimates of the frequency of nonobstetric surgery performed during pregnancy range from 0.3% to 2.2%.1,2 Thus, as many as 93,000 and 110,000 pregnant women in the United States and the European Union, respectively, may require a surgical or anesthetic intervention each year. These numbers are likely to be an underestimation, because pregnancy may be unrecognized at the time of operation. The reported incidence of positive pregnancy tests in women of childbearing age ranged from 0.002% in women presenting for orthopedic surgery3 to 0.3% in women presenting to an ambulatory surgery center4 to 2.6% in women scheduled to undergo elective sterilization procedures.5
The practice of routine pregnancy testing for all women of childbearing age presenting for elective surgery and anesthesia is controversial. The American Society of Anesthesiologists Task Force on Preanesthesia Evaluation states “… the literature is inadequate to inform patients or physicians on whether anesthesia causes harmful effect in early pregnancy. Pregnancy testing may be offered to female patients of childbearing age and for whom the result would change the patient’s management.”6 In 2000, the U.K. National Institute for Health and Clinical Excellence (NICE) issued preoperative screening guidelines recommending routine pregnancy testing for “female patients who say that it is possible they may be pregnant.”7 There was uncertainty among the Guideline Development Group as to whether women should undergo pregnancy testing if the last menstrual period was documented or if women stated that it was not possible that they were pregnant.7 This guideline was poorly followed, resulting in 42 serious incidents, 3 pregnancy losses, and several cases of litigation between 2003 and 2009.8 In 2010, the U.K. National Patient Safety Agency stated that women should be offered a pregnancy test “if there is any possibility that a woman could be pregnant.”8 In certain populations, medical history alone may be an unreliable means of excluding the possibility of pregnancy.6,7 Thus, some institutions routinely perform pregnancy tests on all women of childbearing age who present for elective surgery.3
Surgery may be necessary during any stage of pregnancy. Among 5405 Swedish women who had operations during pregnancy, 42% occurred during the first trimester, 35% during the second trimester, and 23% during the third trimester.1 Laparoscopy for gynecologic indications was the most common first-trimester procedure (34%), whereas appendectomy was the most common procedure during the remainder of pregnancy. Indications for pregnancy-related surgery include cervical incompetence, the presence of ovarian cysts, and conditions amenable to fetal surgery (see Chapter 7). Indications for non–pregnancy-related surgery include the presence of acute abdominal disease (most commonly appendicitis and cholecystitis), malignancies, and trauma.
When caring for pregnant women undergoing nonobstetric surgery, anesthesia providers must consider both the mother and the fetus. Standard anesthetic procedures may have to be modified to accommodate pregnancy-induced maternal physiologic changes and the presence of the fetus. The Confidential Enquiries into Maternal and Child Health in the United Kingdom demonstrate that, even in early pregnancy, mothers die of hemorrhage, sepsis, thromboembolism, and anesthesia; substandard care is often present.9 Erekson et al.10 analyzed 2005 to 2009 data from the American College of Surgeons National Surgical Quality Improvement Program database. The rate of major complications (e.g., infections, reoperation, wound problems, respiratory complications, venous thromboembolism, blood transfusion, maternal death) for antenatal nonobstetric surgery was approximately 6%.10
Possible risks to the fetus of antenatal surgery include (1) the effects of the disease process itself, or related therapy; (2) the teratogenicity of anesthetic agents or other drugs administered during the perioperative period; (3) intraoperative perturbations of uteroplacental perfusion and/or fetal oxygenation; and (4) the risk for abortion or preterm delivery.
Maternal Safety: Altered Maternal Physiology
During pregnancy, profound changes in maternal physiology result from increased concentrations of various hormones, mechanical effects of the gravid uterus, greater metabolic demand, and the hemodynamic consequences of the low-pressure placental circulation. Hormonal changes are likely responsible for most of the changes that occur during the first trimester. Mechanical effects become apparent when the uterus emerges from the pelvis during the second half of gestation (see Chapter 2).
Respiratory System and Acid-Base Balance Changes
Alveolar ventilation increases by 30% or more by mid pregnancy. This increase results in chronic respiratory alkalosis with a PaCO2 of 28 to 32 mm Hg, a slightly alkaline pH (approximately 7.44), and decreased levels of bicarbonate and buffer base. Although oxygen consumption is increased, PaO2 usually increases only slightly or remains within the normal range. Functional residual capacity (FRC) diminishes by approximately 20% as the uterus expands, resulting in decreased oxygen reserve and the potential for airway closure. When FRC is decreased further (e.g., from morbid obesity; perioperative intra-abdominal distention; placement of the patient in the supine, Trendelenburg, or lithotomy position; or induction of anesthesia), airway closure may be sufficient to cause hypoxemia.
Weight gain during pregnancy and capillary engorgement of the respiratory tract mucosa lead to more frequent problems with mask ventilation and tracheal intubation (see Chapter 30). Failed intubation (a leading cause of anesthesia-related maternal death) is as much a risk during early pregnancy with nonobstetric surgery as it is during cesarean delivery.9
Decreased FRC, increased oxygen consumption, and diminished buffering capacity result in the rapid development of hypoxemia and acidosis during periods of hypoventilation or apnea. Moreover, induction of inhalation anesthesia occurs more rapidly during pregnancy because alveolar hyperventilation and decreased FRC allow faster equilibration of inhaled agents. In addition, induction of anesthesia is accelerated owing to the 30% to 40% decrease in the minimum alveolar concentration (MAC) for volatile anesthetic agents that occurs even during early gestation.11 The anesthesia provider must be especially vigilant when administering subanesthetic concentrations of analgesic and anesthetic agents to the pregnant patient, in whom unconsciousness can occur quickly and unexpectedly.
Cardiovascular System Changes
Cardiac output increases by up to 50% during pregnancy because of increases in heart rate and stroke volume; both systemic and pulmonary vascular resistances decrease, but myocardial contractility is unaffected. Early in pregnancy (i.e., by 6 weeks’ gestation), significant cardiovascular alterations are present.12 By 8 weeks’ gestation, 57% of the increase in cardiac output, 78% of the increase in stroke volume, and 90% of the decrease in systemic vascular resistance that are typically achieved by 24 weeks’ gestation have occurred.13
During the second half of gestation, the uterus compresses the inferior vena cava when the mother lies supine; the compression reduces venous return and cardiac output by approximately 30%. Although upper extremity blood pressure may be maintained by compensatory vasoconstriction and tachycardia, uteroplacental perfusion is jeopardized whenever the mother lies supine. In some women, frank hypotension may occur in the supine position, especially when neuraxial or general anesthesia attenuates or abolishes normal compensatory mechanisms. For these reasons, it is essential to displace the uterus laterally during any operation performed after 18 to 20 weeks’ gestation. Vena caval compression also results in distention of the epidural venous plexus, which increases the likelihood of intravascular injection of local anesthetic during the administration of epidural anesthesia. The reduced capacity of the epidural space most likely contributes to the enhanced spread of epidural local anesthetic solution that is observed during pregnancy.
Changes in Blood Volume and Blood Constituents
Blood volume expands in the first trimester and increases 30% to 45% by term. Dilutional anemia occurs as a result of the smaller increase in red blood cell volume than in plasma volume. Although moderate blood loss is well tolerated during pregnancy, preexisting anemia decreases the patient’s reserve when significant hemorrhage occurs. Pregnancy is associated with benign leukocytosis; consequently, the white blood cell count is an unreliable indicator of infection. In general, pregnancy induces a hypercoagulable state, with increases in fibrinogen; factors VII, VIII, X, and XII; and fibrin degradation products. Pregnancy is associated with enhancement of platelet turnover, clotting, and fibrinolysis; there is a wide range in the normal platelet count. Thus, pregnancy represents a state of accelerated but compensated intravascular coagulation. Although thrombocytopenia may be present in some pregnant women, these patients may still be hypercoagulable. During the postoperative period, pregnant surgical patients are at high risk for thromboembolic complications; thus, thromboembolism prophylaxis is recommended.14
Gastrointestinal System Changes
Incompetence of the lower esophageal sphincter and distortion of gastric and pyloric anatomy during pregnancy increase the risk for gastroesophageal reflux, despite similar gastric emptying rates in pregnant and nonpregnant patients15; thus the pregnant woman is at risk for regurgitation of gastric contents and aspiration pneumonitis. It is unclear at what stage during pregnancy this risk becomes significant. Although lower esophageal sphincter tone is impaired early in pregnancy (especially in patients with heartburn),16 the mechanically induced factors do not become relevant until later in pregnancy. It seems prudent to consider any pregnant patient as having a higher risk for aspiration after mid gestation; some anesthesia providers contend that pregnant women are at increased risk for aspiration from the beginning of the second trimester onward.16
Altered Responses to Anesthesia
In addition to the decrease in MAC for inhaled anesthetic agents, thiopental requirements begin to decrease early in pregnancy.17 The effects of pregnancy on propofol requirements are conflicting.18,19 Higuchi et al.18 found that the median effective dose for loss of consciousness (ED50) was unchanged in pregnancy, whereas Mongardon et al.19 reported that lower propofol doses were required to achieve loss of consciousness in early pregnancy compared with the nonpregnant state. This effect appeared unrelated to increased progesterone levels. More extensive neural blockade is attained with epidural and spinal anesthesia in pregnant patients than in nonpregnant patients (see Chapter 12). Pregnancy also enhances the response to peripheral neural blockade.
Plasma cholinesterase levels decrease by approximately 25% from early in pregnancy until the seventh postpartum day. Fortunately, prolonged neuromuscular blockade with succinylcholine is uncommon, because the larger volume available for drug distribution offsets the impact of decreased drug hydrolysis.20 Nonetheless, the dose of succinylcholine should be controlled carefully in the pregnant patient, and the anesthesia provider should monitor neuromuscular blockade with a nerve stimulator to ensure adequate reversal before extubation.
Decreased protein binding associated with lower albumin and alpha-glycoprotein concentrations during pregnancy may result in a larger fraction of unbound drug, with the potential for greater drug toxicity during pregnancy.21 Pregnant surgical patients may require drugs that are infrequently used during pregnancy; limited information may exist on the effects of pregnancy on the response to these drugs. Cautious administration of such agents is advisable, because their pharmacokinetic and pharmacodynamic profiles may differ from those in nonpregnant patients. Pregnancy-associated changes in volume of distribution (due to changes in body composition and/or plasma protein binding capacity), metabolic activity, and hepatic or renal elimination (due to changes in glomerular filtration rate and tubular transport processes) may contribute to changes in drug effects and metabolism.22 For example, in pregnant women, cefazolin clearance is approximately twice as high between 20 and 40 weeks’ gestation and acetaminophen clearance is increased, compared with nonpregnant women.23,24
Fetal Considerations
Risk for Teratogenicity
Although maternal catastrophes that cause severe maternal hypoxia or hypotension pose the greatest risk to the fetus, considerable attention has focused on the role of anesthetic agents as abortifacients and teratogens. Teratogenicity has been defined as any significant postnatal change in function or form in an offspring after prenatal treatment. Concern about the potential harmful effects of anesthetic agents stems from their known effects on mammalian cells, which include reversible decreases in cell motility, prolongation of DNA synthesis, and inhibition of cell division. Despite these concerns, no data specifically link any of these cellular events with teratogenic changes. Unfortunately, prospective clinical studies of the teratogenic effects of anesthetic agents are impractical; such studies would require huge numbers of patients exposed to the drug under investigation. Therefore, investigations of anesthetic agents have taken one of the following directions: (1) studies of the reproductive effects of anesthetic agents in small animals, (2) epidemiologic surveys of operating room personnel constantly exposed to subanesthetic concentrations of inhalation agents, and (3) studies of pregnancy outcome in women who have undergone surgery while pregnant.
Principles of Teratogenicity
A number of important factors influence the teratogenic potential of a substance, including species susceptibility, dose of the substance, duration and timing of exposure, and genetic predisposition. Like other toxicologic phenomena, the effects of teratogens are dose dependent (Figure 17-1).25 Most teratologists accept the principle that any agent can be teratogenic in an animal provided that enough is given at the right time. Thus, the finding of teratogenesis of an agent after the single administration of a high dose, or the long-term administration of a low dose, does not imply that a single, short exposure (e.g., during a typical anesthetic) would incur similar risk. The interaction between dose and timing is also critical. A small dose of a teratogen may cause malformations or death in the susceptible early embryo, whereas much larger doses may prove harmless to the fetus,25 as was shown with thalidomide. Most studies have used small animals (e.g., chick embryos, mice, rats), and their results cannot necessarily be extrapolated to other species, especially humans. Of the more than 2200 agents listed in Shepard’s Catalog of Teratogenic Agents,26 approximately 1200 are teratogenic in animals, but only about 30 of these are known to cause defects in humans.
FIGURE 17-1 Toxic manifestations with increasing dosage of a teratogen. A no-effect range of dosage occurs below the threshold, at which embryotoxic effects abruptly appear. Teratogenesis and embryolethality often have similar thresholds and may increase at roughly parallel rates as dosage increases to a point at which all conceptuses are affected. Increasing dosage causes increased embryolethality, but teratogenicity appears to decrease, because many defective embryos die before term. A further increase in dosage reaches the maternal lethal range. (Modified from Wilson JG. Environment and Birth Defects. New York, Academic Press, 1973:31.)
Manifestations of teratogenicity include death, structural abnormality, growth restriction, and functional deficiency.25 Depending on when it occurs, death is referred to as abortion, fetal death, or stillbirth in humans and as fetal resorption in animals. Structural abnormalities can lead to death if they are severe, although death may occur in the absence of congenital anomalies. Growth restriction is considered a manifestation of teratogenesis and may relate to multiple factors, including placental insufficiency and genetic and environmental factors. Functional deficiencies include a number of behavioral and learning abnormalities, the study of which is called behavioral teratology. The stage of gestation at which exposure occurs determines the target organs or tissues, the types of defects, and the severity of damage. Most structural abnormalities result from exposure during the period of organogenesis, which extends from approximately day 31 to day 71 after the first day of the last menstrual period. Figure 17-2 shows the critical stages of development and the related susceptibility of different organs to teratogens. Functional deficiencies are usually associated with exposure during late pregnancy or even after birth, because the central nervous system (CNS) continues to mature during this period.
FIGURE 17-2 Critical periods in human development. During the first 2 weeks of development, the embryo typically is not susceptible to teratogens. During these predifferentiation stages, a substance either damages all or most cells of the embryo, resulting in its death, or damages only a few cells, allowing the embryo to recover without development of defects. The dark bars denote highly sensitive periods, whereas the light bars indicate periods of lesser sensitivity. The ages shown refer to the actual ages of the embryo and fetus. Clinical estimates of gestational age represent intervals beginning with the first day of the last menstrual period. Because fertilization typically occurs 2 weeks after the first day of the last menstrual period, the reader should add 14 days to the ages shown here to convert to the estimated gestational ages that are used clinically. (Redrawn from Moore KL. The Developing Human. 4th edition. Philadelphia, WB Saunders, 1993:156.)
Consideration of the possible teratogenicity of anesthetic agents must be viewed against the naturally high occurrence of adverse pregnancy outcomes. Roberts and Lowe27 estimated that as many as 80% of human conceptions are ultimately lost; many are lost even before pregnancy is recognized. The incidence of congenital anomalies among humans is approximately 3%, most of which are unexplained. Indeed, exposure to drugs and environmental toxins accounts for only 2% to 3% of such defects (Table 17-1).25 Shepard26 has listed several criteria for determining that an agent is a human teratogen, including the following: (1) proven exposure to the agent at the critical time of development; (2) consistent findings in two or more high-quality epidemiologic studies; (3) careful delineation of the clinical cases, ideally with the identification of a specific defect or syndrome; and (4) an association that “makes biological sense.” Documentation of teratogenicity in experimental animals is important but not essential. The list of agents or factors that are proven human teratogens does not include anesthetic agents (which are listed as “unlikely teratogens”) or any drug routinely used during the course of anesthesia (Box 17-1).
TABLE 17-1
Etiology of Human Developmental Abnormalities
Causes of Developmental Defects in Humans | Percentage |
Genetic transmission | 20 |
Chromosomal aberration | 3-5 |
Environmental causes: | |
Radiation | < 1 |
Infection | 2-3 |
Maternal metabolic imbalance | 1-2 |
Drugs and environmental chemicals | 2-3 |
Unknown | 65-70 |
TOTAL | 100 |
Modified from Wilson JG. Environmental and Birth Defects. New York, Academic Press, 1973:49.
Nondrug Factors Encountered in the Perioperative Period
Derangements of Normal Physiology.
Anesthesia and surgery can cause derangements of maternal physiology that may result in hypoxia, hypercapnia, stress, and abnormalities of temperature and carbohydrate metabolism. These states may be teratogenic themselves, or they may enhance the teratogenicity of other agents.26 Severe hypoglycemia and prolonged hypoxia and hypercarbia have caused congenital anomalies in laboratory animals,26,28 but there is no evidence to support teratogenicity after brief episodes in humans. The chronic hypoxemia experienced by mothers at high altitudes results in the delivery of infants with lower birth weights but with no increase in the rate of congenital defects.26 Maternal stress and anxiety are teratogenic in animals,29 but their significance as human teratogens remains questionable; supporting epidemiologic studies are lacking. Hypothermia is not teratogenic, whereas hyperthermia is teratogenic in both animals and humans.26 Congenital anomalies, especially involving the CNS, have repeatedly been associated with maternal fever during the first half of pregnancy. It must be remembered that fetal temperature is on average 0.5° C to 1° C higher than maternal temperature. Embryonic oxidative stress from reactive oxygen species has been implicated as one of the mechanisms involved in teratogenicity of many agents.30
Diagnostic Procedures.
Ionizing radiation is a human teratogen that can result in an increased, dose-related risk for malignant disease, genetic disease, congenital anomalies, and/or fetal death.26,31 The effects of radiation are often classified as being deterministic or stochastic. Deterministic effects are dose related and are observed above a certain threshold dose (e.g., pregnancy loss, growth restriction, mental retardation, organ malformation). In contrast, stochastic effects are possible at any level of exposure with no minimum threshold but with the likelihood of worsening effects at higher doses. An increased risk for childhood cancer is a stochastic effect when fetuses are exposed to ionizing radiation in utero. The type and severity of effects vary with the radiation exposure to the uterus and fetus and with the gestational age of the fetus.
Radiation is expressed as grays (Gy) or milligrays (mGy) (1 Gy = 100 rad) and is evaluated as cumulative dose (i.e., background radiation and medical diagnostic radiation) throughout the entire pregnancy. Background radiation during gestation is 1.3 to 5.8 mGy.31 There is no evidence that radiation exposure less than 50 mGy is associated with a teratogenic effect in either humans or animals.31 The absorbed fetal dose for all conventional radiographic imaging procedures outside the abdomen and pelvis is negligible and is well below 50 mGy (Table 17-2). However, direct radiographic examination of the abdomen and pelvis (e.g., abdominal computed tomography) and abdominal imaging studies that include fluoroscopy (e.g., barium enema) may result in more significant fetal radiation exposure, with a dose that may approach 100 mGy.31,32 Helical computed tomography for suspected pulmonary embolism results in a lower fetal radiation dose than ventilation perfusion scanning. Interventional radiologic procedures (e.g., cerebral angiography, cerebral embolization, endoscopic retrograde cholangiopancreatography) are frequently complex and prolonged, particularly when surgery is not an option. These procedures, especially the abdominal interventions, expose the fetus to a significant radiation dose; thus, a number of societies have developed guidance for the use of these technologies during pregnancy (Table 17-3).33
TABLE 17-3
Estimated Fetal Radiation Exposure for Common Interventional Radiologic Procedures
Procedure | Estimate (mGy) | Range (mGy) |
Cardiac catheter ablation | 0.15-0.6* | |
ERCP | 3.1 | 0.01-55.9 |
TIPS creation | 5.5 | |
Pulmonary angiography | 0.02-0.46 | |
Uterine fibroid embolization | 42 | |
Cerebral angiography | 0.06 |
* Depending on procedure duration.
ERCP, endoscopic retrograde cholangiopancreatography; TIPS, transjugular intrahepatic portosystemic shunt.
Modified from Dauer LT, Thornton RH, Miller DL, et al. Radiation management for interventions using fluoroscopic or computed tomographic guidance during pregnancy. J Vasc Interv Radiol 2012; 23:19-32.
In contrast to the negligible risk for teratogenicity, observational studies suggest that there is a slightly higher risk for childhood cancer at radiation doses greater than or equal to 10 mGy.32 The relative risk for childhood malignancy after maternal abdominal radiation exposure has been estimated to be 2.28 (95% confidence interval, 1.31 to 3.97).31 Nonetheless, if the mother’s condition necessitates diagnostic testing and radiation exposure, and no other acceptable imaging modality is available, testing should not be withheld if the benefits to the mother (and, by extension, the fetus) are judged to outweigh the risks. Delayed diagnosis of a critical condition may result in significant harm to the mother and, by extension, to the fetus. The radiographer should adhere to the ALARA principle (as low as reasonably achievable) and take measures to minimize the absorbed dose of radiation, including abdominal shielding, reduced scan length, and milliampere modulation.
Intravenous iodinated contrast media crosses the placenta to the fetus when administered in the usual clinical doses and can potentially cause neonatal hypothyroidism; however, the use of radiopaque (iodide containing) contrast media during pregnancy is acceptable.34
Diagnostic ultrasonography during pregnancy has long been considered to be devoid of embryotoxic effects. In animals, ultrasound intensities up to 20 W/cm2 have been found to be safe.35,36 However, when higher intensities (> 30 W/cm2) have been used, or when repeated exposure has occurred during early pregnancy, postnatal neurobehavioral effects have been described.36,37 Ultrasound waves also increase the fetal temperature, and hyperthermia is a known teratogen. Modern diagnostic ultrasound equipment is capable of inducing significant increases in temperature, especially when imaging is prolonged. Miller et al.38 concluded that these temperature increments were within the range of temperatures that could be teratogenic. Epidemiologic human data are reassuring; no biologic effects have been documented from diagnostic ultrasonographic examinations in the pregnant patient, despite widespread use over several decades. Nonetheless, it must be stressed that these epidemiologic studies were conducted in an era when ultrasound equipment was less potent and ultrasound-related increases in temperature were lower. Doppler interrogation emits significantly more acoustic intensity than pulse-echo imaging equipment; thus, more heat is generated. Therefore, Doppler technology should be used judiciously, keeping the exposure time and acoustic output to the lowest level possible.
No adverse effects on pregnancy and neonatal outcome were noted in a small series of pregnant patients (n = 26) exposed to gadolinium (paramagnetic contrast medium).39 However, gadolinium-based MRI contrast agents readily cross the placenta, enter the fetal circulation, and are excreted by the fetal kidneys into the amniotic fluid, where they may be swallowed by the fetus or resorbed by the mother. Although no toxic effects have been described after gadolinium administration, the long-term consequences remain unknown. The American College of Radiology recommends that intravenous gadolinium be avoided during pregnancy and used only if absolutely essential.40
Systemic Agents
Animal Studies.
Early studies documented teratogenicity in rodents after a variety of neurotropic agents (e.g., opioids, tricyclic antidepressants).41,42 The fetuses exhibited a characteristic group of CNS malformations as well as skeletal abnormalities and growth restriction. Whether these investigations truly reflect the teratogenic potential of opioids is questionable, however, because the respiratory depression and impaired feeding that accompany large bolus injections of opioids also may be teratogenic. In a study designed to avoid such problems, Fujinaga and Mazze43 maintained clinically relevant concentrations of morphine throughout most of pregnancy in rats by means of continuously implanted osmotic mini-pumps. Structural anomalies were not observed at any morphine dose, although fetal growth restriction was present, and mortality was increased among the offspring. Using the same methodology, Fujinaga et al.44,45 found fentanyl, sufentanil, and alfentanil completely devoid of teratogenic effects. Additional animal studies have confirmed the absence of teratogenicity with other opioids.46
Many tranquilizers and anxiolytics taken by pregnant women have been investigated less systematically than opioids. Animal studies have demonstrated structural or behavioral teratogenesis after exposure to some of the barbiturate, phenothiazine, and tricyclic antidepressant agents.47,48 The reader is referred to standard teratology reference sources for animal data related to specific drugs.26,49 The package insert provided by a drug’s manufacturer typically describes unpublished in-house studies related to the drug’s reproductive effects.
Studies in rodents and nonhuman primates indicate that exposure of the immature brain to anesthetic agents such as propofol, thiopental, and ketamine (i.e., agents classified as N-methyl-D-aspartate [NMDA] antagonists and gamma-aminobutyric acid [GABA] agonists)] are associated with brain cell apoptosis and functional learning deficits.50,51 Whether this effect occurs in humans or other species remains to be determined (see Chapter 10). These observed changes may represent indirect effects, such as hypoxia or hypoglycemia, as well as direct effects of the anesthetic agent on the developing brain.
Human Studies.
Teratogenesis has not been associated with the use of any of the commonly used induction agents—including the barbiturates, ketamine, and the benzodiazepines—when they were administered in clinical doses during anesthesia.26 Similarly, no evidence supports the teratogenicity of opioids in humans; there is no increase in the incidence of congenital anomalies among offspring of mothers who use morphine or methadone during pregnancy.26,49
Although human data relating to long-term tranquilizer therapy have raised questions about the possible teratogenicity of some agents, most studies are retrospective and suffer from a variety of methodologic flaws. Benzodiazepine therapy became controversial after an association between maternal diazepam ingestion during the first trimester and infants with cleft palate, with or without cleft lip, was reported.52 Subsequently, prospective work in 854 women who ingested diazepam during the first trimester did not demonstrate a higher risk associated with benzodiazepine therapy.53 Although the present consensus among teratologists is that diazepam is not a proven human teratogen,26 it is appropriate to consider the risk-to-benefit ratio before initiating long-term benzodiazepine therapy during the first trimester. No evidence suggests that a single dose of a benzodiazepine (e.g., midazolam) during the course of anesthesia is harmful to the fetus.
Local Anesthetics
Procaine, lidocaine, and bupivacaine cause reversible cytotoxic effects in cultures of hamster fibroblasts.54 However, no evidence supports morphologic or behavioral teratogenicity associated with lidocaine administration in rats,55 and no evidence supports teratogenicity associated with any local anesthetic used clinically in humans.26 Maternal cocaine abuse is associated with adverse reproductive outcomes, including abnormal neonatal behavior and, in some reports, a higher incidence of congenital defects of the genitourinary and gastrointestinal tracts.26 The greatest risk to the fetus most likely results from the high incidence of placental abruption associated with maternal cocaine use (see Chapter 54).
Muscle Relaxants
Testing muscle relaxants for teratogenicity using standard in vivo animal studies either is complicated by maternal respiratory depression and the need for mechanical ventilation (a complex undertaking in rats or mice) or requires the administration of very low doses of the drug. Fujinaga et al.56 used the whole-embryo rat culture system to investigate the reproductive toxicity of high doses of D-tubocurarine, pancuronium, atracurium, and vecuronium. Although dose-dependent toxicity was manifested, these effects occurred only at concentrations 30-fold greater than those encountered in clinical practice. These findings are consistent with earlier studies demonstrating no toxicity with small doses of muscle relaxants.57 Given that fetal blood concentrations of muscle relaxants are only 10% to 20% of maternal concentrations, these drugs appear to have a wide margin of safety when administered to the mother during organogenesis.
Whether their administration later in gestation has adverse effects is unclear. Prolonged disturbance of normal muscular activity by muscle relaxants has caused axial and limb deformities in the chick but has seldom been seen in other experimental animals. Although one case report described arthrogryposis (i.e., persistent joint flexure) in the infant of a woman with tetanus who received D-tubocurarine for 19 days beginning at 55 days’ gestation, the patient also was hypoxic and received multiple other drugs.58 Many women have received muscle relaxants for several days during late gestation without adverse effect on the neonate.
Inhalation Anesthetics
Animal Studies.
Volatile Agents.
Many studies have shown that, under certain conditions, the volatile halogenated anesthetic agents can produce teratogenic changes in chicks or small rodents. Basford and Fink59 observed skeletal abnormalities but no increase in fetal loss when rats were exposed in utero to 0.8% halothane for 12 hours on days 8 and 9 of pregnancy (i.e., the “critical period” in the 21-day rat gestation). Long-term exposure to subanesthetic concentrations of halothane caused fetal growth restriction in rats but no increase in the incidence of congenital anomalies,60 whereas isoflurane had no adverse effects.61
More significant reproductive effects have occurred with greater exposures to anesthetic agents. Fetal skeletal abnormalities or death followed repeated or prolonged maternal exposure of mice to anesthetic concentrations of volatile anesthetic agents.62 However, teratogenicity in these studies most likely was caused by the physiologic changes (e.g., profound hypothermia, hypoventilation) associated with anesthesia rather than by the anesthetic agent itself. Moreover, some strains of mice are especially likely to demonstrate anomalies such as cleft palate. Mazze et al.63 exposed rats to 0.75 MAC of halothane, isoflurane, or enflurane, or 0.55 MAC of nitrous oxide for 6 hours daily on 3 consecutive days at various stages of pregnancy. The animals remained conscious throughout the study, and normal feeding and sleep patterns were preserved. Under these conditions, no teratogenic effects were associated with any of the volatile agents. The only positive finding was a threefold increase in the rate of fetal resorption with nitrous oxide. No evidence has suggested reproductive toxicity with either sevoflurane or desflurane in clinical concentrations.
Nitrous Oxide.
In contrast to the volatile halogenated agents, nitrous oxide is a weak teratogen in rodents under certain conditions, even when normal homeostasis is maintained. Rats continually exposed to 50% to 70% nitrous oxide for 2 to 6 days (starting on day 8 of gestation) had an increased incidence of congenital abnormalities.63,64 To exclude the possibility that adverse effects were a consequence of the anesthetic state, Lane et al.65 exposed rats to 70% nitrous oxide or to a similar concentration of xenon (a slightly more potent anesthetic devoid of biochemical effects) for 24 hours on day 9 of gestation; abnormalities occurred only in the nitrous oxide group. With the exception of one study in which extremely prolonged exposure to a low concentration of nitrous oxide had some minor effects,66 at least 50% nitrous oxide has been required to consistently produce anomalies.64 The threshold exposure time has not been rigorously determined, although exposure for at least 24 hours typically was necessary.
In vivo and embryo culture studies in rats have confirmed that nitrous oxide has several adverse reproductive effects, each of which results from exposure at a specific period of susceptibility.67,68 Fetal resorptions occurred after exposure on days 8 and 11 of gestation, skeletal anomalies after exposure on day 8 or 9, and visceral anomalies (including situs inversus) only when exposure occurred on day 8.69
Initially, teratogenicity associated with nitrous oxide was thought to result from its oxidation of vitamin B12, which interferes with its function as a coenzyme for methionine synthase.70 Transmethylation from methyltetrahydrofolate to homocysteine to produce tetrahydrofolate (THF) and methionine is catalyzed by methionine synthase (Figure 17-3). Thus, methionine synthase inhibition could cause a decrease in THF (with a resultant decrease in DNA synthesis) and lower methionine levels (with resultant impairment of methylation reactions). Nitrous oxide rapidly inactivates methionine synthase in both animals71 and humans.72 Prolonged human exposure to nitrous oxide leads to neurologic and hematologic symptoms, the latter probably resulting from diminished DNA synthesis.70 The hematologic—but not the neurologic—changes are prevented by the co-administration of folinic acid (5-formyl THF) with nitrous oxide, with the goal of restoring DNA synthesis.
FIGURE 17-3 Pathway showing the inhibition of methionine synthase by nitrous oxide (N2O) and its potential metabolic consequences (e.g., decreased DNA synthesis and impaired methylation reactions). THF, tetrahydrofolate. (Courtesy M. Fujinaga, Palo Alto, CA.)
Considerable evidence indicates that methionine synthase inhibition and a consequent lack of THF are not solely responsible for the teratogenic effects of nitrous oxide. First, maximal inhibition of methionine synthase activity occurs at concentrations of nitrous oxide that are much lower than those required to produce teratogenic effects.73 Second, folinic acid, which bypasses the effect of methionine synthase inhibition on THF formation, partially prevents only one of the structural abnormalities (i.e., minor skeletal defects) produced by nitrous oxide.74 Third, the administration of isoflurane or halothane with nitrous oxide prevents almost all of the teratogenic effects but does not prevent the decrease in methionine synthase activity.75,76 Fourth, studies using an in vitro whole-embryo rat culture system have shown that supplementation of nitrous oxide with methionine (but not with folinic acid) almost completely prevents growth restriction and all malformations with the exception of situs inversus.77 Additional studies have implicated α1-adrenergic receptor stimulation in the production of situs inversus by nitrous oxide.68,78,79 Postulated mechanisms by which sympathetic stimulation might have adverse reproductive effects include a decrease in uterine blood flow and overstimulation of G protein–dependent membrane signal transduction pathways.80 There is also evidence that nitrous oxide may cause neuronal apoptosis in rats.81
In summary, evidence suggests that the etiology of nitrous oxide teratogenicity in rats is complex and multifactorial. Determination of the relative roles of methionine deficiency and sympathetic stimulation or other mechanisms awaits performance of further studies. Although nitrous oxide is considered a weak teratogen in rats and mice, reproductive effects occur only after prolonged exposure to high concentrations that are unlikely to be encountered in humans during clinical anesthesia. Whether nitrous oxide administration is associated with neuronal apoptosis and learning impairments in humans remains to be determined.
Human Studies.
Occupational Exposure to Waste Anesthetic Agents.
Epidemiologic surveys dating from the 1960s and 1970s suggested that reproductive hazards (e.g., spontaneous abortion, congenital anomalies) were associated with operating room and dental surgery work.82–86
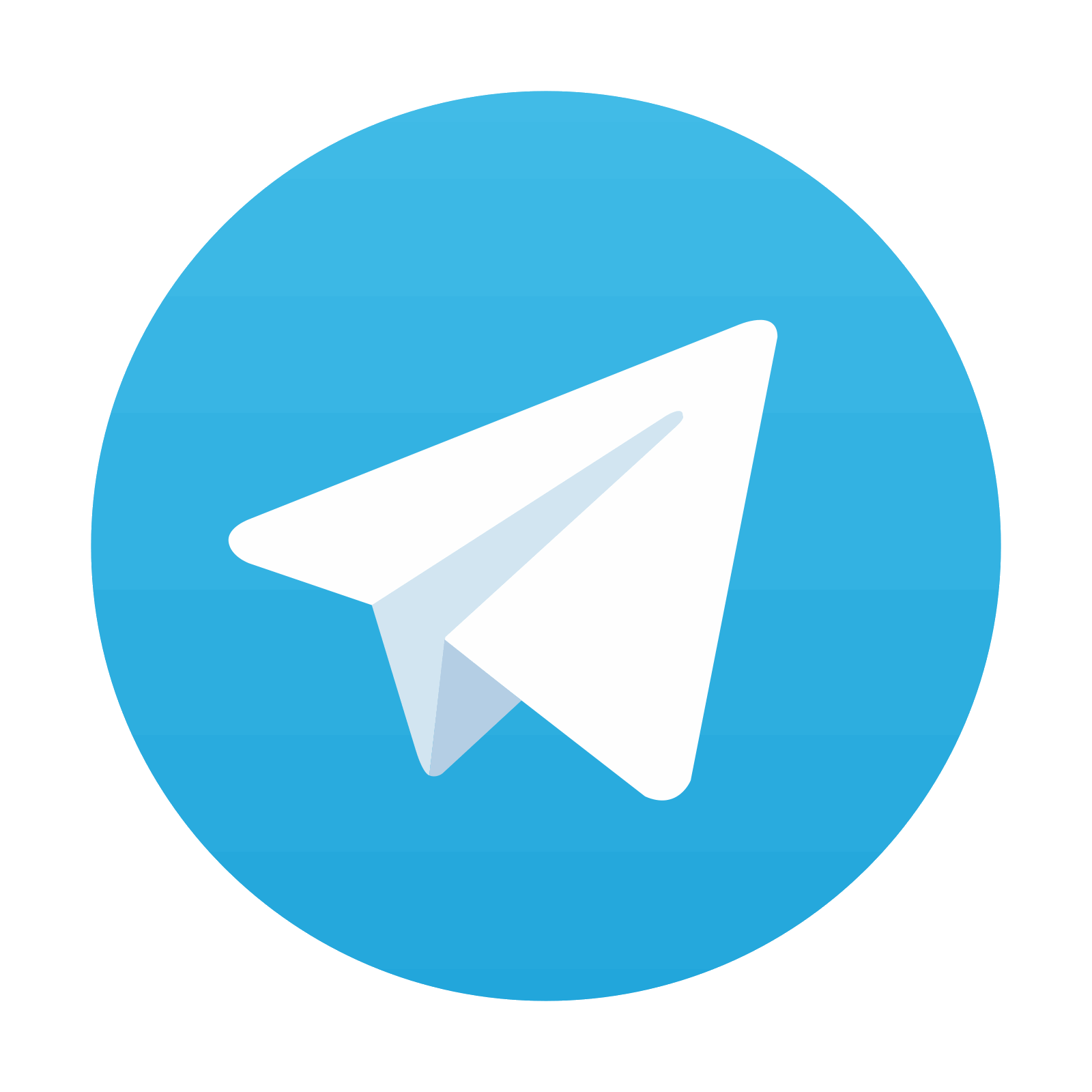
Stay updated, free articles. Join our Telegram channel
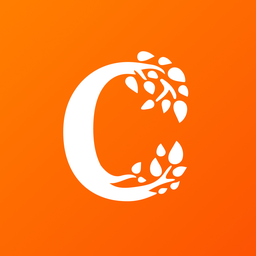
Full access? Get Clinical Tree
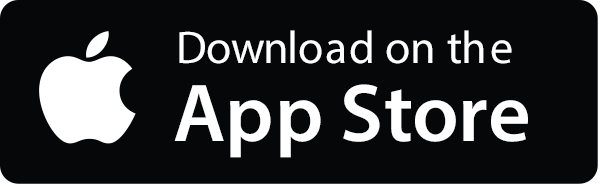
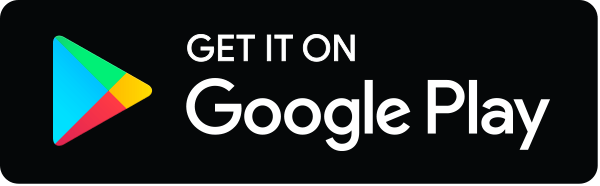