Fig. 21.1
Fetal adaptive response to hypoxia and hypercapnia. Compensative mechanisms work in the initial phase through an increase in cerebral blood flow. If the insult persists, neuronal damage is due to the loss of these mechanisms
In this scenario, seizures and abnormal movements such as clonus or tremors are correlated to an impaired synaptic glutamate uptake in the brain. Since glutamate is an excitatory neurotransmitter, there is a subsequent overactivation of its excitatory postsynaptic receptors (AMPA, NMDA, and kainate) with intracellular accumulation of Na+ and Ca++.
There is a primary energy failure phase that occurs at the cellular level due to the loss of readily available oxygen to the brain. For this reason, cellular metabolism shifts to anaerobic, and this reliance upon anaerobic metabolism pathways leads to depletion of adenosine triphosphate (ATP) and a lack of energy from enzymes such as Na+/K+-ATPase, with a subsequent rapid cytotoxic edema and necrotic cell death (Fig. 21.2).

Fig. 21.2
Excitatory postsynaptic effect due to impairment of glutamate uptake, resulting in increased intracellular Na+ and Ca++, associated with a reduced function of the Na+/K+-ATPase and cellular damage
After an initial phase asphyxial damage, cerebral metabolism may reactivate, with the establishment of regular blood perfusion. This event leads to a secondary damage, called “delayed phase neuronal damage” that starts at about 6–24 h after the initial damage and is characterized by mitochondrial dysfunction and apoptotic damage. Neonatal seizures typically occur in this phase. The delayed phase damage duration is not precisely known in the fetus and in human infants, but appears to increase in the first 24–48 h and is correlated with a poor prognosis of neurodevelopment at 1 and 4 years after the insult [24].
During the reperfusion period, enzymes such as cyclooxygenase, xanthine oxidase, and lipoxygenase increase the production of free radicals, resulting in damage to the brain of the newborn, damages that are amplified by the immaturity of his antioxidant defenses.
The increase of free radicals can lead to lipid peroxidation as well as to damage of DNA and proteins and can trigger apoptosis. Finally, free radicals can combine with nitric oxide (NO) which production has an early and transient increase. NO concentration observed in the initial phase of hypoxia is due to the activation of NMDA receptors and neuronal nitric oxide synthases (NOS). The excessive production of NO plays an important role in the pathophysiology of ischemic perinatal hypoxic hypoxia [25]. Its neurotoxicity depends in large part on the rapid reaction with superoxide to form peroxynitrite which is the cause of lipid peroxidation, nitration and protein oxidation, mitochondrial damage, and DNA damage [25] (Fig. 21.3).

Fig. 21.3
Delayed phase neuronal damage after reperfusion period and restart of cerebral metabolism. The oxidative stress is not adequately opposed by an immature antioxidant system of the fetal brain. ROS reactive oxygen species, NO nitric oxide
In addition, the existence of a third phase where deleterious factors may cause further damage and potentiate neuronal injury worsening neonatal outcomes has been recently proposed. This third phase is thought to include mechanisms of inflammation and epigenetic changes that lead to an impairment or alteration of axonal growth, neurogenesis, and synaptogenesis [26].
21.4 Clinical Signs associated with Neonatal Hypoxic-Ischemic Encephalopathy
The clinical symptoms of HIE start early, at birth, or in the first hours of life. The newborn with HIE may show an abnormal state of consciousness (irritability, lethargy, stupor, coma), reduction in spontaneous movements, difficulties in breathing or nutrition, reduced tone, abnormal posture, lack of primitive reflexes, or seizures. HIE is the major underlying cause of seizures in term infants, with a large variety of clinical presentations from subtle, clonic, tonic, and myoclonic, potentially ranging from focal, multifocal, or generalized. Status epilepticus is common as well as an involvement of other organs (liver, kidney, heart).
In the delivery room, the Apgar score is generally low and crying is weak or absent. The severity of neonatal encephalopathy can be classified as mild, moderate, or severe, depending on the clinical symptoms and on the Sarnat three-stage grading system, as follows [16, 17, 27, 28]:
- (a)
Mild encephalopathy: it is characterized by irritability, increased muscle tone, and accentuation of deep tendon reflexes during the first days of life. They may also present transitional behavioral abnormalities, such as poor nutrition, excessive weeping, or absence of sleep. These symptoms usually resolve within 24–48 hours after birth.
- (b)
Moderate encephalopathy: it is associated with lethargy, significant hypotonia, and reduced deep tendon reflexes. The reflexes such as Moro and suction can be reduced or absent. There may be occasional periods of apnea and seizures within the first 24 hours after birth.The recovery may occur within 1 to 2 weeks after birth.
- (c)
Severe hypoxic-ischemic encephalopathy: seizures are usually generalized with onset in the 24–48 hours after birth, correlating with the reperfusion phase. Generalized hypotonia is associated with depressed tendon reflexes and absent neonatal reflexes (suction, swallowing, grasping, Moro). A state of stupor or coma is typically present, so the child does not respond to the external stimuli except to the most harmful. Examination of the cranial nerves may reveal abnormalities such as altered eye motion (distorted deviation of the eyes, nystagmus, and loss of doll’s eye movement, pupils may be dilated, fixed, or poorly reactive to light). In case of recurrent apnea, the infant often requires ventilatory support.
21.5 Diagnosis of Neonatal Encephalopathy
The American College of Obstetricians and Gynecologists (ACOG) recommends a comprehensive evaluation in all cases of neonatal encephalopathy [1]. This evaluation should include an assessment of neonatal clinical status and consideration of all factors potentially contributing to neonatal encephalopathy, including a thorough maternal and family history, focusing on thromboembolic disorders, maternal infection, maternal drug use, obstetric antecedents, and intrapartum factors (including fetal heart rate monitoring results and issues related to delivery).
Blood samples should be used to determine umbilical artery cord pH and base deficit. The presence of oliguria, cardiomyopathy, or abnormal liver function tests may suggest a global hypoxic-ischemic event. In addition, a gross and histologic examination of the placenta and umbilical cord may provide evidence of a possible cause, such as a placental vascular lesion or infection, or an umbilical cord thrombosis [24]. The presence of neonatal dysmorphic features and congenital anomalies may suggest the presence of an inborn error of metabolism or genetic disorder.
21.5.1 Neuroimaging in the Workup for Neonatal Encephalopathy
Neuroimaging is a useful tool to provide information regarding the type and timing of brain injury, since there are certain distributional patterns of brain injury seen in term and late preterm infants that are considered to be typical of hypoxic-ischemic brain injury [29–31].
The findings on CT and MRI of the brain may provide clues regarding the time during which the injury occurred [32, 33].
Other modalities of neuroimaging have been used to evaluate brains of infants with neonatal encephalopathy, including cranial sonography, and MRI with magnetic resonance spectroscopy.
Among these techniques, MRI yielded the most useful information.
21.5.1.1 Neonatal MRI
Neonatal MRI is rapidly becoming a standard of care at tertiary care centers in the United States and some other developed countries. It is the most sensitive imaging tool for detecting cortical and white matter injury, deep gray matter lesions, arterial infarction, hemorrhage, developmental brain malformations, and other underlying causes of neonatal encephalopathy [29, 30, 34–37].
Injury to the deep gray nuclei (especially the lateral thalami and posterior putamina) [16, 34, 38, 39] corresponds to brain damage seen in animal models of acute total asphyxia [40] and has been found in 74 % of term neonates with hypoxic encephalopathy associated with a sentinel event [41].
Deep gray matter lesions involving the bilateral basal ganglia and thalami are particularly common findings on brain MRI in encephalopathic term infants with a recognized preceding sentinel hypoxic-ischemic event such as placental abruption, uterine rupture, or umbilical cord prolapse [41]. Brainstem injury may also be common in these circumstances [2, 42].
Parasagittal injury of the cerebral cortex and subcortical white matter in the arterial watershed distribution may occur in the setting of mild hypoxia or ischemia of prolonged or chronic duration.
Conventional MRI may be of limited value in the early phases, so the use of advanced techniques, such as magnetic resonance spectroscopy or diffusion-weighted imaging, has been utilized to detect early injury [37, 43].
Magnetic resonance spectroscopy allows in vivo quantitative analysis of brain metabolites and therefore may serve as an early biomarker for brain injury. Indeed, an elevated ratio of lactate to N-acetyl aspartate in the basal ganglia can predict long-term neurologic impairments and can be seen in the first 48 h of life [37].
Diffusion-weighted imaging measures the self-diffusion of water molecules detected as an apparent diffusion coefficient (ADC). Thus, the ADC decreases with acute injury and is reflective of reduced water diffusion in the tissue. However, during the first hours after the injury, this technique may underestimate the final extent of the injury [37].
Studies of adult arterial infarcts have shown that diffusion-weighted imaging signal changes occur within minutes from symptom onset and hours before changes become apparent on T1- or T2-weighted images. This earlier detection of injury may facilitate intervention prior to irreversible injury [43].
Alternatively, a brain imaging study may reveal a developmental malformation, focal arterial infarction, or intraparenchymal hemorrhage, indicating a different underlying pathogenesis for the neonatal encephalopathy, venous infarction, isolated intraparenchymal or intraventricular hemorrhage, and porencephaly.
21.5.1.2 Cranial Sonography
Cranial sonography ultrasound has the advantage of being noninvasive, easily repeatable and less expensive. It has a high sensitivity and specificity (91 % and 81 %, respectively) for detecting hemorrhages and ventricular size [44, 45]. It may also detect severe parasagittal white matter damage and obvious cystic lesions, but it does not adequately describe the outer limits of the cerebral cortex [46] as well as milder white matter abnormalities [47].
Cranial sonography can be used to detect severe cerebral edema, appearing as diffuse increased echogenicity that causes sulci and fissures to be obscured, blurring of other anatomical landmarks, decreased arterial pulsations, and compression of the cerebral ventricles [48, 49].
Although in early neonatal period, areas of increased echogenicity correspond to regions of necrosis, it is not always possible to establish an exact correlation between these images and infarction or hemorrhage in the term neonatal brain [50]. However, Doppler sonography, when used for assessment of neonatal hypoxia, may identify a decrease in refractive indexes in arteries due to a relative increase in diastolic flow velocities. A number of other limitations of sonography for the diagnosis of hypoxic-ischemic injury in the term neonate have been identified. These include a substantial percentage of false negative, a low sensitivity for detecting cortical lesions, and dependence on the skill of the operator performing the test [37].
21.5.1.3 Neonatal Brain Computed Tomography (CT)
Computed tomography is more sensitive than cranial sonography for diagnosing intracranial hemorrhage [30] but is also a valuable diagnostic tool in identification of cerebral edema, cerebral atrophy, abnormal ventricular size, and severe white matter lesions [51–54]. Since the white matter in a term newborn brain has a high water content, CT is less sensitive in the identification of milder degree edema and white matter injury.
Radiation exposure is a limiting factor in its use in small infants [30], although CT is more feasible than MRI for children who are acutely ill or medically unstable.
21.5.2 Electroencephalography (EEG)
EEG is a tool to evaluate the severity of brain damage in neonates with HIE. This technique supplies information on the functional aspects of the central nervous system.
An EEG can help to distinguish neonatal seizures from other phenomena and can also identify subclinical seizures. Due to difficulties in the provision of expert interpretation of conventional multichannel EEG in the neonatal intensive care units, amplitude-integrated EEG is more commonly used.
Amplitude-integrated EEG using a continuous, single- or dual-channel recording of background cerebral electrical activity is easy to use and interpret at the bedside and has been used to distinguish mild from severe neonatal encephalopathy in large clinical trials [55, 56].
An abnormal EEG is one of the necessary criteria to enroll infants with HIE in brain cooling treatment [57].
21.6 Definition of Hypoxic-Ischemic Encephalopathy
Hypoxic-ischemic encephalopathy (HIE, also called birth asphyxia) is a subset of neonatal encephalopathy, but determining whether an acute hypoxic-ischemic event contributed to neonatal encephalopathy is challenging, since there is no gold standard test for diagnosis. In order to consider a HIE as a possible cause of an acute perinatal asphyxial damage, the following symptoms and signs have been suggested by the American College of Obstetricians and Gynecologists (ACOG) and the American Pediatric Association (APA) [1]: pH <7, metabolic acidosis, Apgar score 0–3 for over 5-min period, neonatal neurological sequelae (convulsions, coma, hypotonia), and multisystem involvement.
A pH of the cord artery >7.2 is hardly associated with HIE, although the arterial blood gas of the cord alone is not sufficiently accurate in predicting long-term neurological sequelae [58, 59].
A pH <7.0 is the threshold for a clinically significant acidemia [60]. The incidence of adverse neurological outcomes is described as 0.36 % with a neonatal pH <7.1 and 3 % with a pH <7.0 [59], although many acidemic infants will be neurologically normal [61].
A base deficit ≥12 mmol/L increases the possibility that a neonatal encephalopathy is caused by HIE. In fact, the encephalopathy develops in 10 % of infants in which the base deficit of the umbilical artery is between 12 and 16 mmol/L, and 40 % of those whose deficit is >16 mmol/L [62].
In addition, lactate concentration in the umbilical cord may have a higher value compared to base deficit for the prognosis of neurological disorders [63].
A low (<5) Apgar score at 5 and 10 min is associated with increased risk of neurological damage, and this risk is higher if the Apgar scores at 5 min are 3 or less [58].
The persistence of an extremely low (0–3) Apgar score over 5 min after birth strongly correlates with increased risk of neurologic morbidity and death [64, 65].
Finally, an Apgar score of 0 at 10 min is correlated to a high mortality rate or serious long-term disability [66].
21.7 Treatment of Neonatal Encephalopathy
Therapeutic hypothermia is considered the standard of care for neonates with HIE.
This treatment employs mild hypothermia in the range of 33.5–35.0 °C, maintained for 72 hours and started within the first 6 hours after delivery, as the only effective neuroprotective therapy currently available for treatment of neonatal encephalopathy for term or late preterm infants.
Potential mechanisms of neuroprotection with hypothermia include (a) inhibition of glutamate release, (b) reduction of cerebral metabolism which in turn preserves high energy phosphates, (c) decrease in intracellular acidosis and lactic acid accumulation, (d) preservation of endogenous antioxidants, (e) reduction of nitric oxide production, (f) prevention of protein kinase inhibition, (g) improvement of protein synthesis, (h) reduction of leukotriene production, (i) prevention of blood-brain barrier disruption and brain edema, and (j) inhibition of apoptosis. Therapeutic hypothermia can reduce also seizures and epileptiform activity burden in term newborns with moderate HIE [67, 68].
Treatment with hypothermia improves survival and outcome at 18 months after neonatal asphyxia and/or neonatal encephalopathy. This conclusion is supported by a meta-analysis of seven randomized controlled trials of therapeutic hypothermia involving 1,214 newborns with moderate to severe neonatal encephalopathy [69].
It should be associated with a supportive management of moderate and severe neonatal encephalopathy which should take place in a neonatal intensive care unit. Major goals include the maintenance of physiologic homeostasis and treatment of the manifestations of brain injury [2, 70]. Central aspects of supportive care include (1) maintenance of adequate ventilation (avoidance of hypoxemia or hyperoxia), (2) maintenance of sufficient brain and organ perfusion (avoidance of systemic hypotension or hypertension, avoidance of hyperviscosity), (3) maintenance of normal metabolic status (normoglycemia, nutritional status, pH), (4) control of seizures, and (5) brain edema, avoiding fluid overload.
Therapeutic hypothermia is easy to administer and appears to be safe. Although direct comparisons are lacking, selective head cooling and whole body cooling appear to have similar safety and effectiveness. Whole body cooling is preferred in most centers in the United States due to ease of administration. Whole body cooling also provides easier access to the scalp for electroencephalogram (EEG) monitoring.
In general, eligibility criteria include the following [71]:
- (a)
Gestational age ≥35 weeks and ≤6 hours of age
- (b)
One of the following:
-
A 10-min Apgar score of <5
-
Ongoing resuscitation (assisted ventilation, chest compressions, or cardiac medications) initiated at birth and continued for at least 10 min
-
pH of ≤7.0 or a base deficit of ≥16 mmol/L in a sample of umbilical cord blood or any blood obtained within the first hour after birth
-
- (c)
Moderate to severe encephalopathy on clinical examination (indicated by lethargy, stupor, or coma) and either hypotonia, abnormal reflexes (including oculomotor or pupillary abnormalities), an absent or weak suction, or clinical seizures
- (d)
Abnormal background activity of at least 30-min duration or seizures on EEG or amplitude-integrated EEG
When therapeutic hypothermia is not used, a close monitoring of body temperature is suggested, and it is reasonable to avoid hyperthermia given currently available data. There is a consensus among experts that therapeutic hypothermia should be more widely available, based upon its benefit and safety and the lack of other effective treatments [72–74]. Thus, hypothermia has become the standard of care in most neonatal intensive care units in the United States, Europe, Australia, and Japan, and national guidelines support the use of therapeutic hypothermia for infants who meet the criteria used in the published trials [75–77].
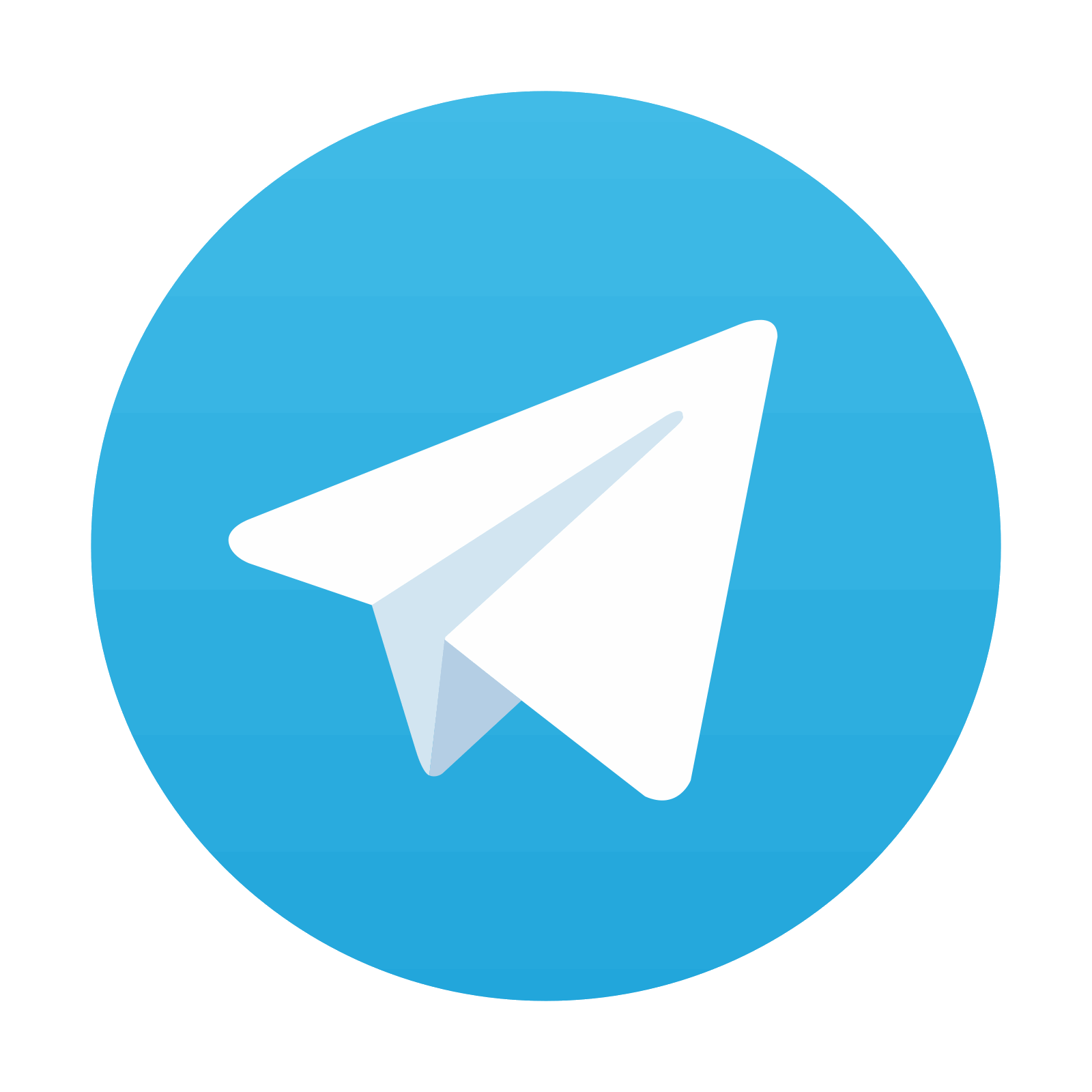
Stay updated, free articles. Join our Telegram channel
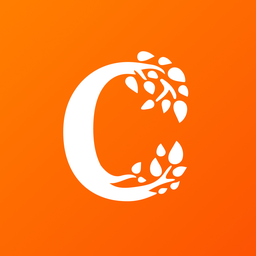
Full access? Get Clinical Tree
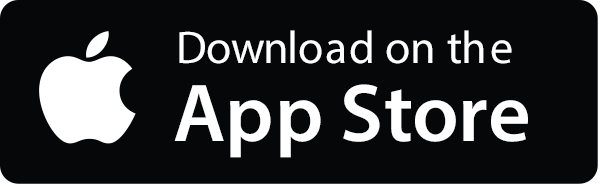
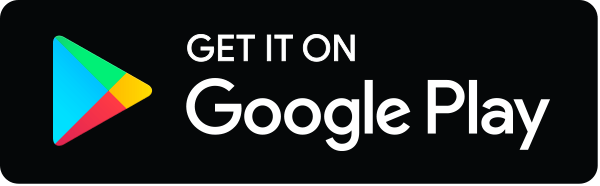