10.2 Modern genetics
A clinical geneticist is a medical specialist who has expert knowledge of human clinical and laboratory genetics. However, because genetic information underlies the development and responses of every individual, an understanding of human genetics is essential for every medical practitioner. This chapter provides a brief overview of the principles of genetics as they pertain to paediatrics, but readers are cautioned that there is much that is both important and fascinating that cannot be covered in the space available. It is strongly recommended that readers utilize other resources that are relevant to this section. Readers should also bear in mind that genetics is a rapidly developing field, and detailed information derived from any source may become outdated within a matter of months.
Many technical terms are used throughout this chapter and readers need to be familiar with these terms. Many will be familiar from courses in genetics or biochemistry.
Genetic information
Genetic information is encoded in DNA
Deoxyribonucleic acid (DNA) is a complex molecule located within the nucleus of every cell of a body (Fig. 10.2.1.) It is the biological ‘library’ of the genetic information needed for a fertilized egg to develop into a complex and functional organism (a person) comprised of a wide variety of cell and tissue types.
• DNA is a linear molecule comprised of subunits called nucleotides.
• There are four different nucleotides; adenosine (A), thymidine (T), cytosine (C) and guanidine (G).
• Each nucleotide is linked to its immediate neighbour by a sugar molecule, deoxyribose, forming a long chain.
• Two chains of nucleotides are linked together by hydrogen bonds.
• Adenosine in one chain pairs with thymidine in the other chain, and cytosine pairs with guanidine.
• The hydrogen bonds between the A–T and C–G pairs are like the rungs on a ladder.
• The entire ladder is twisted along its length into a conformation that is often called a ‘double helix’.
• The entire DNA sequence is called the human genome and is encoded by approximately three billion (3 × 109) nucleotides.
DNA is located in two different parts of a cell
The human genome is separated into two parts. The smaller mitochondrial genome (mtDNA) is located in the matrix of mitochondria and encodes just 37 genes. The larger nuclear genome (nuclear DNA) is located in the nucleus of the cell and encodes more than 25 000 genes.
Nuclear DNA forms tightly coiled lengths of DNA called chromosomes
Nuclear DNA is packaged into 23 fragments of varying length. During mitosis each fragment is visible down a light microscope as a short bundle or chromosome. A gene is located at the same point on the same chromosome in all human individuals. A locus is the unique chromosome position that defines the location of each individual gene or DNA sequence.
• Human somatic (‘body’) cells have two copies of the nuclear genome, one copy inherited from each parent; this DNA is packaged into 46 chromosomes (23 pairs).
• Sperm and ova (gametes) have a single copy of the nuclear genome packaged as 23 unpaired chromosomes.
• Fertilization of an ovum by a sperm restores the usual amount of nuclear DNA in a somatic cell; 46 chromosomes; 23 pairs.
• In a karyotype, chromosome pairs are arranged in a standard format based on their size, banding pattern and centromere position; the first 22 pairs are called autosomes; the 23rd pair are the two sex chromosomes.
• In females there are two equivalent long sex chromosomes called X chromosomes.
• In males there is one long X chromosome and a small Y chromosome.
One X chromosome is inactivated in females
The difference in the number of X chromosomes between males and females represents a profound difference in the amount of genetic information in the cell. Females compensate for the presence of two X chromosomes by inactivating one X chromosome, a process called lyonization.
• At the time of conception both X chromosomes in a female conceptus are active.
• After a few cell divisions, one X chromosome in each cell is inactivated by methylation; the same X chromosome remains inactive in all daughter cells derived from that ancestral cell.
• The result is that both males and females have only one active X chromosome in each cell.
• On average, each of the two X chromosomes will be active in half of the cells of a female’s body.
• Because X inactivation is initiated when the conceptus consists of a small number of cells, by chance approximately 10% of females have the same X chromosomes active in 90% or more of cells (skewed X inactivation).
Mitochondrial DNA is a small, circular, double-stranded DNA
Mitochondria are small organelles within the cell that have an essential role in many metabolic processes including oxidative phosphorylation, the process that generates adenosine triphosphate (ATP) via aerobic metabolism. Mitochondria contain more than 1000 different proteins, including over 60 proteins directly involved in oxidative phosphorylation. Most, but not all, of these mitochondrial proteins are encoded by nuclear genes.
• mtDNA is a double-stranded loop, 16 569 nucleotides long, that is joined end-to-end in a circle.
• mtDNA encodes 13 proteins involved in oxidative phosphorylation, as well as 24 non-coding ribonucleic acids (RNAs) important for normal mitochondrial function.
• Each mitochondrion has 5 to 10 copies of mtDNA and, depending on the type of tissue, a single somatic cell may contain as many as 1000 mitochondria.
• Tissues that are highly dependent on oxidative phosphorylation (e.g. neurons) have more mitochondria per cell than tissues that are less dependent (e.g. some epithelial cells).
• Mitochondria and mtDNA are copied independently of the process of copying nuclear DNA.
• Mitochondria are maternally inherited – all the mitochondria in an individual’s cells are derived from the original ovum.
• The exclusive maternal inheritance of the mitochondrial DNA is in marked contrast to the bi-parental inheritance of nuclear DNA.
The function of our genome: DNA, genes and proteins
Protein-coding genes
A unit of DNA sequence that encodes a protein is called a gene. The genetic information contained in the gene flows from DNA to RNA to protein.
• A gene consists of a sequence of nucleotides.
• Some genes are encoded by several hundreds of nucleotides and others by many thousands.
• The DNA sequence of most nuclear genes is broken up into coding modules (exons), which are separated from each other by regions of non-coding DNA (introns) (Fig. 10.2.2).
• Mitochondrial genes do not have introns.
• Specific DNA sequences define the beginning and the end of a gene.
• Exons are usually a few hundred nucleotides long.
• Introns may be many thousands of nucleotides in length.
• Introns have regulatory and evolutionary roles that are poorly understood.
Non-protein-coding genes
A unit of DNA sequence that encodes a functional, non-coding RNA transcript is also called a gene.
The DNA sequence of a gene is transcribed into RNA
To access the genetic information encoded in a gene, the enzyme RNA polymerase uses the gene’s nucleotide sequence as a template to synthesize a molecule of RNA, a process called transcription (see Fig. 10.2.2).
• RNA has a structure that is similar to DNA, except it is usually single-stranded and uracil (U) substitutes for thymidine.
• DNA is transcribed to a primary RNA transcript; a series of processing reactions follow, including removal of introns, producing a shorter, mature RNA transcript.
• There are many different types of mature RNA that have a range of different functions, some unknown.
• Protein-coding genes encode a special form of mature RNA, messenger RNA (mRNA), which serves as a template for synthesis of a protein polypeptide.
mRNA is translated into protein via a triplet nucleotide code
Protein polypeptides are made of many amino acids joined end to end in a long chain with a specific sequence. This chain of amino acids folds to form a three-dimensional shape that is unique to each specific protein polypeptide.
• mRNA is translated (decoded) to make a protein polypeptide at ribosomes.
• The information encoded in the mRNA sequence is interpreted via a triplet nucleotide code (a codon), which determines the exact amino acid sequence of the protein (see Fig. 10.2.2).
• There are also specific stop codons that indicate the end of a gene.
Our understanding of the function of DNA is changing
Until recently the accepted understanding was that genes occur infrequently along the human genome and that these genes encode RNA, which in turn encodes the amino acid sequence of protein polypeptides. By this view, a gene encodes a single protein polypeptide and is separated from its neighbouring genes by long stretches of intergenic non-coding ‘junk’ DNA. It is now clear that this is a simplified interpretation of the function of DNA, and of what constitutes a gene. Below is a summary of our current understanding of the function of the genome, but readers are again cautioned that this information will rapidly become outdated and that other resources should be consulted.
• About 5% of the human genome sequence has been highly conserved by evolution, and most of this DNA is functionally important.
• Around one-fifth of this conserved sequence encodes proteins (protein-coding DNA).
• The other four-fifths of this conserved sequence is non-protein-coding DNA which encodes functionally important RNA molecules that are not translated into proteins (i.e. non-coding RNA transcripts).
• Although the remaining 95% of the human genome sequence is less conserved, it is estimated that around 85% is transcribed to non-coding RNA transcripts.
Gene regulation
The regulation of human gene expression is incompletely understood
Our understanding of the ways the human genome is regulated and integrated within a cell (or an individual) is rudimentary. It is beyond the scope of this chapter to discuss all that is known or hypothesized about gene regulation, but the reader should be familiar with two important concepts: inactivation of genes by methylation, and imprinting.
Genes are controlled by regulatory DNA sequences. These regulatory sequences can be chemically altered by the addition of methyl groups (− CH3) to the nucleotides of the sequence, this is called methylation.
• Methyl groups interfere with the binding of molecules to the regulatory sequence of a gene.
• The net effect of this methylation is inactivation of the gene.
• Methyl groups may also be removed, activating a gene.
• Methylation provides a means for varying gene activity in normal cells.
• Some genes are inactivated in cells of a specific tissue type(s), resulting in tissue-specific expression of the gene.
• Some genes are inactivated at certain times during development leading to developmental stage-dependent expression.
• X chromosome inactivation in females is an example of gene inactivation due to methylation.
The activation or silencing of a gene is usually independent of whether the gene was inherited paternally or maternally. An exception is genes that are imprinted.
• Imprinting is the selective inactivation of a gene according to the sex of the parent who passed on that gene.
• Imprinting is a normal process and results in only one active copy of the gene in a cell.
• Inactivation occurs because of methylation of a regulatory region of the gene.
• Some imprinted genes are selectively inactivated when transmitted by sperm and selectively activated when transmitted by an ovum (they are paternally imprinted).
• Other genes demonstrate the reciprocal pattern and are selectively inactivated when transmitted by an ovum and selectively activated when transmitted by a sperm (they are maternally imprinted).
• Normally the methylation ‘imprint’ is removed in sperm and ova so that inactivation of the imprinted gene is always determined by the sex of the parent of origin.
• Imprinting is an example of an epigenetic mechanism; a heritable change in gene expression that does not occur because of a change in DNA sequence.
Failure of normal imprinting results in overexpression or underexpression of a gene, which may lead to a clinical disorder.
• Failure to imprint a gene results in there being two active copies of the gene, producing a ‘functional duplication’ of that gene.
• Inappropriate imprinting of a gene results in there being two inactive copies of the gene, producing a ‘functional deletion’ of that gene.
• Examples of imprinting disorders include Prader–Willi syndrome, Angelman syndrome, Russell–Silver syndrome and Beckwith–Wiedermann syndrome.
Uniparental disomy
Uniparental disomy is the situation where a child inherits two copies of a particular chromosome from one parent and none from the other, rather than having one copy from each parent. The total number of chromosomes is normal, but if any gene(s) on the chromosome is normally imprinted a functional genetic imbalance results.
When the paternal copy of the gene is normally imprinted:
• if both copies of the gene are inherited from the father there are zero active copies of the gene instead of the normal single active copy and a ‘functional deletion’
• if both copies of the gene are inherited from the mother there are two active copies of the gene and a ‘functional duplication’.
When the maternal copy of the gene is normally imprinted:
Genes and networks
A single gene does not produce its effect independent of the action of other genes. All processes within a single cell are regulated by an interacting network of many genes. For example, a network of several hundred different genes is involved in intracellular galactose metabolism. This leads to a degree of biological robustness because a mutation (genetic error) in a single gene will not necessarily result in cellular dysfunction if the remainder of the genetic network is able to compensate for the mutated gene.
This network of genes is embedded within a network of interacting cells within a single tissue or organ, which is embedded in a network of interacting tissues and organs within a single individual. Individuals, in turn, are embedded in social and environmental networks. The interplay and variation within and between these genetic, cellular, biological, social and environmental networks helps explain why the clinical features of a specific disorder can vary from one individual to another.
Cystic fibrosis (CF) is a common autosomal recessive disorder:
• It affects approximately 1 in 2500 Caucasian children.
• 1 in 25 healthy Caucasian adults are carriers of a mutation that can cause CF.
• More than 2000 different mutations have been identified in the CF gene (genetic heterogeneity).
• One mutation (deltaF508) accounts for about 80% of all mutations; other mutations are much less common.
• Without treatment, children with classical CF usually die during infancy.
• With treatment, at least 50% of these children are alive in their mid-40s.
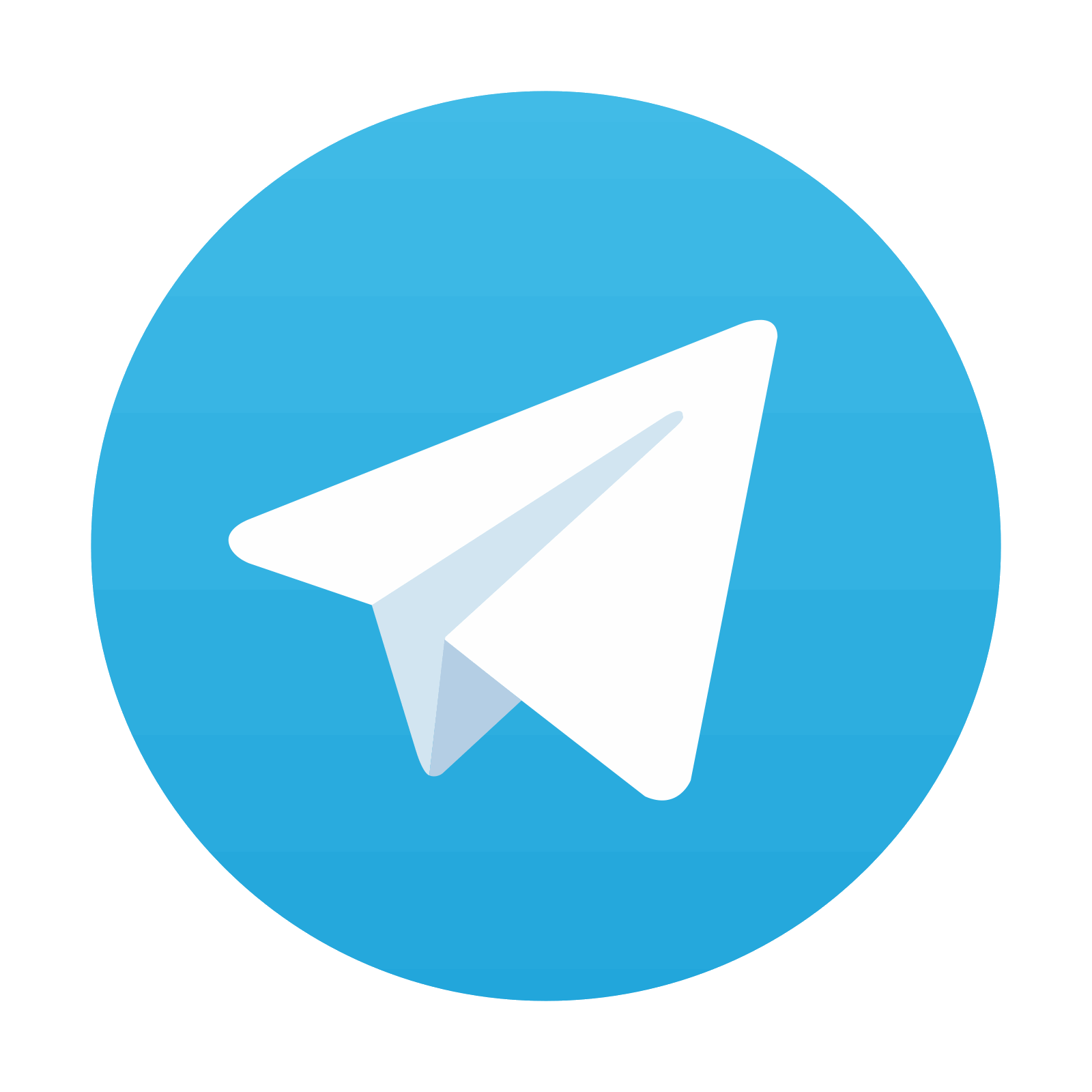
Stay updated, free articles. Join our Telegram channel
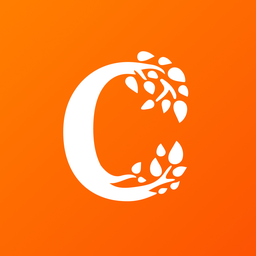
Full access? Get Clinical Tree
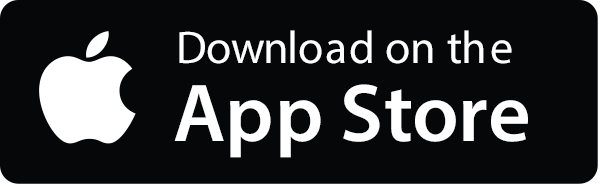
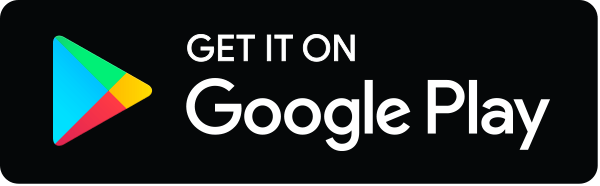