Key Points
- •
Survival at birth depends upon the lung attaining an adequate size and degree of structural maturity during fetal life. This chapter deals with mechanisms underlying normal and impaired lung growth and lung maturation before birth.
- •
The airways of the fetal lung contain a liquid that is actively secreted by the epithelium; this ‘lung liquid’ causes the lung to develop in an expanded state, which is necessary for normal lung growth and maturation.
- •
The degree of lung expansion in a fetus is determined by the lung’s physical environment, including intrathoracic space, fetal breathing movements (FBMs) and amniotic fluid volume. Mechanical stress in lung tissue stimulates gene networks, leading to tissue growth and differentiation. The long-term absence of the physiological stretch stimulus leads to lung hypoplasia.
- •
Clearance of lung liquid begins with the onset of labour caused by (i) imposed fetal postural changes that cause loss of lung liquid via the nose and mouth and (ii) active reabsorption across the lung epithelium. Luminal liquid remaining after birth is cleared because of transpulmonary pressure gradients generated by inspiration.
- •
Pulmonary blood flow (PBF) is generally low during fetal life but can increase with FBMs. At birth, pulmonary vascular resistance decreases markedly, thereby permitting increased blood flow through the lungs, which is necessary for adequate gas exchange.
- •
Lung aeration at birth underpins the cardiovascular transition at birth, including the marked increase in PBF. With the loss of umbilical venous return at birth, the increase in pulmonary venous return takes over the critical role of supplying preload for the left ventricle.
- •
Maturation of the lung in preparation for birth involves extracellular matrix remodelling, alveolar epithelial cell differentiation and surfactant production. These changes are driven by mechanical stress in lung tissue and corticosteroid signalling.
Introduction
In fetal life, the lungs play no role in gas exchange, but at birth, they must immediately take over from the placenta the critical role of gas exchange. This transition is normally uneventful, which is remarkable given that before birth, the lungs are liquid filled with a low blood flow. For the lung to function as an organ of gas exchange at birth, it must cease the secretion of fetal lung liquid, and the airways must be cleared of luminal liquid; the lungs must produce adequate amounts of surfactant; and pulmonary vascular resistance (PVR) must be reduced, allowing them to receive the entire output of the right ventricle. During normal fetal development, the lung becomes progressively prepared for these dramatic changes in physiology at birth. However, if lung growth or maturation in utero is impaired or if an infant is born before term, the newborn infant may develop respiratory distress syndrome (RDS). This chapter focuses on the processes controlling prenatal lung growth and maturation and highlights the physiological changes that underpin the transition to newborn life. Some of the more common respiratory complications in neonates and their fetal origins are discussed together with strategies for their treatment.
Stages of Lung Development
Pulmonary morphologists recognise five or six major stages in human lung development ( Table 11.1 ).
Stage | Gestational Age | Major Events |
---|---|---|
Embryonic | 4–7 wk | Appearance of ventral bud in foregut. Epithelial tube branches and grows into surrounding mesenchyme. Vascular connections formed. |
Pseudoglandular | 5–17 wk | Development of bronchial tree, paralleled by formation of vascular tree. Lung periphery contains parenchymal precursors. |
Canalicular | 16–26 wk | Addition of further generations of airways and vascular tree. Differentiation of type I and type II epithelial cells. Formation of thin air–blood barrier. Start of surfactant production. |
Saccular stage | 25–40 wk (term) | Formation of additional airway generations. Dilation of prospective gas-exchanging airspaces. Maturation of surfactant system. |
Alveolar stage | 36 wk–18 mo | Start of alveolar formation by outgrowth of secondary septa. |
Microvascular maturation | Birth–3 yr | Change from double- to single-capillary network. Reduction in interstitial tissue mass; fusion of capillaries; preferential growth of single-layered capillary network areas. |
Embryonic Stage (4–7 Weeks)
The lung first appears as an outgrowth of the primitive foregut (i.e., endodermal tissue) at 22 to 26 days postconception. This bud divides to form the left and right bronchi, which then undergo dichotomous branching to form the major units of the bronchial tree. During early embryonic development, epithelial cells that are endodermal in origin form the developing ‘airways’ and grow into the surrounding tissue, which is derived from splanchnic mesoderm. This mesodermal tissue gives rise to the mesenchymal cells that ultimately form the nonepithelial structures of the lung, including blood and lymph vessels, airway cartilage and smooth muscle, fibrous tissue and other components of the lung parenchyma.
Pseudoglandular Stage (5–17 Weeks)
During the pseudoglandular stage, the lung resembles a typical exocrine gland. The major bronchi and associated functional units of the lung (i.e., acini) progressively form, accompanied by branches of the pulmonary arterial tree. As a result, each major ‘airway’ is accompanied by a branch of the pulmonary artery. The formation of each acinus (respiratory unit) results from repeated branching of the distal extremities of blind-ending tubes or ‘airways’ composed of epithelial cells ( Fig. 11.1 ). This process of branching (branching morphogenesis) is induced by airway epithelial cells interacting with adjacent mesenchymal cells, which are supplied by a loose network of capillaries (see Fig. 11.1 ). Airway epithelial cells gradually differentiate (in a centrifugal direction) into specific cell types: ciliated cells (by 11–13 weeks), goblet cells and mucous glands.

Canalicular Stage (16–26 Weeks)
During the canalicular stage, the airways widen and lengthen, and mesenchymal tissue surrounding the distal airways becomes attenuated (see Fig. 11.1 ). This process (canalisation) results in a substantial increase in the ratio of lumen volume to tissue volume. During the canalicular stage, the functional units of the lung are formed, consisting of terminal bronchioles ending with expansions that subsequently form terminal sacs (primitive alveoli). A network of blood capillaries develops around the terminal air sacs, increasing the proximity of blood capillaries to the epithelial surface; this marks the beginning of the air–blood interface that is required for effective gas exchange (see Fig. 11.1 ). Thus the late canalicular stage is the earliest at which the lungs can support independent life.
Terminal Sac Stage (28–40 Weeks)
The terminal sac, or saccular, stage of lung development sees a progressive enlargement of the distal ‘air spaces’. This enlargement results from further attenuation of perisaccular mesenchymal tissue and leads to a further increase in luminal volume relative to lung tissue volume. During the terminal sac stage, the development of secondary septa begins; these outgrowths from primary septa will eventually subdivide the terminal sac into multiple alveoli (see Fig. 11.1 ). The primitive primary septa, which separate adjacent saccules, are thicker than secondary septa and contain a double capillary network rather than the single capillary layer of the mature alveolus. Elastic fibres are formed by myofibroblasts within secondary septa and are deposited at their tips, thereby contributing to the inherent elastic (recoil) properties of the lung. The epithelial cells become differentiated into type I and type II epithelial cells. As a result of these structural changes, the separation between luminal ‘air’ and capillary blood becomes smaller, thereby enhancing the ability of the lung to exchange respiratory gases after birth.
Alveolar Stage (36 Weeks of Gestation to 1–2 Years)
During the alveolar stage, terminal sacs become subdivided by the outgrowth of secondary septa from the primary septa to form alveoli. Initially, these alveoli resemble shallow cups, but they deepen because of elongation of the secondary septa. The alveolar walls and the epithelial cells lining them become thinner, leading to the formation of definitive alveoli. The mean alveolar diameter increases greatly, from about 30 μm at 30 weeks to about 150 μm at 40 weeks. The final stage of alveolar maturation involves the restructuring of the capillary network, such that the more primitive double capillary network lining the terminal sacs and alveoli is transformed into a single layer of capillaries (see Fig. 11.1 ); this marks the existence of definitive alveoli.
By the time of term birth, the human lung contains 20 to 50 million alveoli. An adult human lung contains approximately 300 million alveoli, indicating that most are formed postnatally. The alveolar stage of lung development is thought to continue for at least 1 to 2 years after birth, although some alveoli may continue to be formed later in life. In species born at an earlier stage of development (e.g., rats and mice), the alveolar stage begins after birth; therefore, at birth, gas exchange occurs across terminal sacs.
Pulmonary Circulation
Structural Development
The structural development of the pulmonary vasculature has recently been reviewed in detail. The lung develops with two anatomically and functionally distinct vascular systems: the pulmonary system, which supplies the gas-exchanging tissue (alveoli), and the bronchial system, which perfuses the non–gas-exchanging tissue of the lung. The arteries of the bronchial circulation give rise to capillaries which supply the walls of the bronchi and bronchioles but do not extend to the most peripheral gas-exchanging parts of the bronchial tree; bronchial venous blood returns via the pulmonary veins because of anastomoses between the bronchiolar and pulmonary veins. The pulmonary arteries develop a muscular wall except near the lung periphery, where the arteries are only partially muscularised. Pulmonary veins show a branching pattern similar to that of arteries but do not follow the arteries and airways; rather, they tend to run at right angles in the mesenchyme.
Arterioles are virtually absent in the adult pulmonary circulation; therefore, pulmonary blood flow (PBF) is determined largely by the resistance of the alveolar capillaries. In fetuses and newborns, however, the smooth muscle surrounding the small pulmonary arteries is thicker than in an adult lung, relative to diameter, and extends farther down the vascular tree. This likely contributes to the high vascular resistance of the fetal lung and may be a consequence of the high fetal pulmonary artery pressure (relative to postnatally). In the first few weeks after birth, the arterial smooth muscle thins, leading to a reduction in arterial wall thickness, likely because of a reduction in pulmonary arterial pressure following the functional separation of the pulmonary and systemic circulations.
The creation of an efficient gas exchange surface within the lung depends upon the development of a dense capillary bed in close proximity to the epithelium of the terminal sacs or alveoli. Early in development, capillaries form a loose network adjacent to the immature airspaces, but the two structures are usually separated by other cells and extracellular matrix (ECM) components. During the canalicular stage, the number of capillaries increases greatly, and they come into close contact with the epithelium of the primitive air sacs. By the terminal sac stage, the capillaries form a dense network around these sacs and, with further attenuation of tissue between adjacent sacs, the basement membrane underlying the capillary endothelial cells fuses with the basement membrane underlying the alveolar epithelial cells ( Fig. 11.2 ). The sites of basement membrane fusion are initially focal, but they expand as the lung matures, providing a very thin (∼0.2 μm) barrier for gas exchange.

Functional Development of the Pulmonary Circulation
This topic has recently been reviewed in depth. At midgestation, only 3% to 4% of total cardiac output perfuses the lung, and by late gestation, this has risen to only 8% to 10%. In a fetus, most (∼88%) of the right ventricular output is diverted away from the lungs to the descending aorta via the ductus arteriosus. Mean pulmonary arterial pressure in a near-term fetus is about 55 mm Hg, which is about 5 mm Hg higher than mean aortic pressure, thereby maintaining flow from the pulmonary to the systemic circulation through the ductus arteriosus. Although PVR declines progressively during fetal life due to a large increase in total cross-sectional area of the pulmonary vascular bed, it remains much higher (up to eightfold) just before birth than immediately after birth.
In fetuses, PVR is influenced by a range of factors, including the physical and oxygen environments of pulmonary vessels and the presence of vasoactive agents. Because this topic has been extensively reviewed, only a brief outline will be provided here. In fetal sheep, PVR is reduced by vigorous fetal breathing movements (FBMs) and is closely related to lung liquid volume. Increasing the volume, and hence pressure, of liquid within the terminal air sacs likely compresses the small pulmonary vessels (mainly capillaries), thereby increasing PVR. The low P o 2 of blood perfusing the fetal lungs also contributes to a high PVR. In postnatal lambs, perfusion of the lungs with blood having a P o 2 similar to in fetuses greatly increases PVR. In fetal sheep, lowering and raising the P o 2 of arterial blood (i.e., hypoxia and hyperoxia) causes increases and decreases in PVR, respectively. The mechanism by which oxygen tension influences PVR is unknown but may involve the release of prostacyclin (PGI 2 ) and endothelium-derived nitric oxide (NO), both of which affect vascular smooth muscle.
Changes at Birth
Lung aeration is the primary trigger for the decrease in PVR at birth, resulting in an 8- to 10-fold increase in PBF. Although the increase in PBF is enhanced by increased oxygenation, it is not dependent on an increase in oxygenation and occurs even if the lungs are ventilated with 100% nitrogen. Furthermore, as partial lung aeration leads to a global increase in PBF, increasing equally in both aerated and nonaerated regions ( Fig. 11.3 ), the increase in PBF is not spatially related to aerated lung regions. This can lead to a large ventilation/perfusion mismatch in the lung if it only partially aerates at birth, which as argued later, is likely to be more beneficial than harmful for the transitioning infant.

The mechanisms underlying the large fall in PVR at birth are complex and probably involve alterations in the physical environment, the oxygen environment and the balance between vasodilator and vasoconstrictor substances. Because the alveolar epithelium and capillary endothelium are mechanically coupled (see Fig. 11.2 ), the creation at birth of an air–liquid interface in the alveoli and the partial recoil of the lungs (see later) likely alter the geometry of small pulmonary vessels, causing them to dilate. Rhythmic expansion of the lungs at birth, increased oxygenation and release of vasodilators such as PGI 2 , bradykinin (a potent vasodilator in the fetus) and NO, are thought to mediate pulmonary vasodilation after birth. Indeed, inhibition of NO synthesis before birth attenuates the birth-induced increase in PBF and results in pulmonary hypertension in the newborn.
None of the above-mentioned mechanisms can readily explain the global increase in PBF induced by partial lung aeration because the increase occurs equally in aerated and nonaerated lung regions and can be induced by ventilation with 100% N 2 (see Fig. 11.3 ). Although the mechanism is unknown, it has been suggested that the clearance of airway liquid into the surrounding perialveolar tissue could activate J-receptors which signal via vagal afferents to effect global pulmonary vasodilation.
The increase in PBF at birth plays a critical role in the cardiovascular transition at birth. Before birth, because PBF is low, preload for the left ventricle is predominantly derived from umbilical venous return, which flows via the ductus venosus, inferior vena cava and foramen ovale to enter the left atrium. Thus if the umbilical cord is clamped before the lungs have aerated and PBF has increased, the left ventricle will be deprived of preload until the lungs aerate and PBF increases. This can cause a sustained decrease in cardiac output (by up to 50%) after birth and places the infant at high risk for systemic hypotension and hypoxic-ischemic brain injury. This is because an increase and redistribution of cardiac output are the primary mechanisms that protect the brain from oxygen deficiency during hypoxic-asphyxic episodes. On the other hand, aerating the lung and increasing PBF before the umbilical cord is clamped allows the supply of preload blood to the left ventricle to immediately switch from umbilical venous to pulmonary venous return without any decrease in supply. As a result, the characteristic decrease in cardiac output (often evidenced as a decrease in heart rate) is prevented when the onset of ventilation precedes umbilical cord clamping. Furthermore, in view of the vital role that a high PBF plays after birth in providing preload for the left ventricle and maintaining cardiac output, it is logical for the increase in PBF to be independent of, and not linked (at least initially), to the degree of lung aeration.
Fetal Breathing Movements
Episodes of breathing-like movements occur intermittently throughout much of gestation in healthy mammalian fetuses. These movements, termed ‘fetal breathing movements’, share key features with postnatal breathing and are thought to be an early expression of coordinated, rhythmical activity in the brainstem regions responsible for the control of breathing. FBMs involve rhythmic ‘inspiratory’ activation of the diaphragm and dilator muscles of the larynx. Expiratory muscles (e.g., intercostal muscles, upper airway constrictors) are not significantly active during periods of unstimulated FBM. Similar to postnatal breathing, FBMs are stimulated by increased arterial PaCO 2 and by decreased pH in the blood and cerebrospinal fluid, probably via stimulation of central chemoreceptors. FBMs are also influenced by fetal behavioural states; they principally occur in association with a state resembling rapid eye movement (REM) sleep and are absent during the state resembling quiet (slow-wave or non-REM sleep. Whether the fetus is ever in a state of wakefulness is controversial; however, short periods of a fetal state of heightened activity resembling wakefulness (low-voltage electrocortical activity, eye movements, fetal swallowing, head and neck movements) are usually accompanied by FBM. The suppression of FBM during fetal quiet (non-REM) sleep is of considerable interest because this suppression must cease after birth when breathing becomes continuous, regardless of sleep–wake states. It is currently thought that breathing becomes continuous after birth as a result of increased CO 2 production and removal from putative inhibitory agents released by the placenta.
Fetal breathing movements differ from postnatal breathing in that the ‘tidal volume’ is very small, and each ‘breath’ is essentially isovolumic; the low tidal volume of a fetus can be attributed to the high viscosity (and inertia) of water relative to air, and the high resistance of moving liquid through the respiratory tract. In ovine fetuses, the ‘tidal volume’ is normally less than 1 mL compared with 40 to 50 mL (8–10 mL/kg) in newborn lambs ; a similar difference exists in humans. That is, the change in lung luminal volume associated with individual FBM in late gestation is normally less than 1% of resting (baseline) luminal volume. Thus FBM resemble postnatal breathing with an obstructed upper airway; this presumably gives rise to the paradoxical nature of FBM during which the chest wall retracts during inspiratory efforts and the abdominal wall moves out.
Fetal breathing movements are readily detected by ultrasound and, as a component of the fetal biophysical profile, have been used to assess fetal health. The incidence of FBMs is reduced in fetuses that are subjected to intrauterine stresses such as hypoxemia, hypoglycaemia, intrauterine infection, increased levels of prostaglandins and labour. FBMs may be absent or impaired in fetuses with congenital abnormalities of the nervous system or skeletal muscle. Maternal drug use can also abolish or attenuate FBM; for example, alcohol consumption, tobacco smoking and common sedatives and narcotics can inhibit FBM.
Fetal breathing movements are now known to be critical for normal lung development; indeed, the prolonged absence of FBM results in hypoplastic lungs, in which the lungs are small and structurally immature. If severe, lung hypoplasia can result in respiratory insufficiency or death in the newborn. FBMs play a critical role in maintaining the fetal lungs in an expanded state by opposing the inherent tendency of the fetal lung to recoil. Lung expansion during fetal life is known to be necessary for the normal growth and structural maturation of the lungs; the role of FBMs in maintaining lung expansion is discussed in more detail later.
Fetal Lung Liquid
Control of Fetal Lung Liquid Secretion
The future air spaces of the fetal lung contain a liquid that is secreted by the pulmonary epithelium. This unique liquid (fetal lung liquid) is not inhaled amniotic fluid because it accumulates in the lungs when the trachea is obstructed, and it has an ionic composition very different to that of amniotic fluid ( Table 11.2 ). Measurements of unidirectional ion fluxes in fetal sheep lungs indicate that lung liquid secretion results from the net movement of chloride and sodium ions across the pulmonary epithelium into the ‘airway’ lumen. This generates a transepithelial osmotic gradient that promotes the movement of water into the lung lumen. It is thought that Na + ,K + -ATPase, located on the basolateral surface of pulmonary epithelial cells, provides the electrochemical gradient for Na + to enter the cell coupled to Cl – . The Cl – then exits the cell across its apical membrane and enters the lung lumen down the transmembrane electrochemical gradient. The net movement of Cl – into the lung lumen provides an electrical gradient for Na + to enter the lumen as well; together these ionic movements create an osmotic gradient for the movement of water from the cell into the lung lumen. After being secreted, fetal lung liquid leaves the lung via the trachea and enters the pharynx, from where it is either swallowed or enters the amniotic sac.
Parameter | Arterial Blood | Lung Liquid | Amniotic Fluid |
---|---|---|---|
Na + (mmol/L) | 150 ± 0.7 | 150 ± 1.3 | 113 ± 6.5 |
K + (mmol/L) | 4.8 ± 0.2 | 6.3 ± 0.7 | 7.6 ± 0.8 |
Cl – (mmol/L) | 107 ± 1.0 | 157.1 ± 4.1 | 87 ± 5 |
Ca 2+ (mmol/L) | 3.3 ± 0.1 | 0.8 ± 0.1 | 1.6 ± 0.1 |
HCO 3 − (mmol/L) | 24 ± 1.2 | 2.8 ± 0.3 | 19 ± 3 |
Urea | 291 ± 2 | 7.9 ± 2.7 | 10.5 ± 2.4 |
Osmolality (mOsm) | 291 ± 2 | 294 ± 2 | 265 ± 2 |
Protein (mg/100 mL) | 4090 ± 260 | 27 ± 2 | 100 ± 10 |
pH | 7.34 ± 0.04 | 6.27 ± 0.5 | 7.02 ± 0.09 |
Although there are no data on lung liquid secretion for human fetuses, fetal sheep secrete lung liquid at 3 to 4 mL/kg/h during the last third of gestation before the onset of labour. The rate of secretion is controlled by endocrine, metabolic and physical factors. Both adrenaline, acting via β-adrenergic receptors, and arginine vasopressin are potent inhibitors of lung liquid secretion in vivo , possibly via activation of adenylate cyclase leading to an increase in intracellular cyclic adenosine monophosphate (cAMP) concentrations. Other hormones known to affect fetal lung liquid secretion include cortisol and prolactin, both of which increases lung liquid secretion in vivo.
Because lung liquid secretion is an active process, it is inhibited by hypoxaemia (acute or chronic), an effect which is likely to be mediated by a reduction in oxygen availability or associated changes in tissue pH rather than an increase in circulating adrenaline and vasopressin. Physical factors such as the pulmonary luminal liquid pressure also influence the secretion of fetal lung liquid. A reduction in fetal lung liquid volume, and hence a reduction in luminal pressure, increases lung liquid secretion rates. In contrast, sustained increases in fetal lung expansion, which increase luminal pressure, lead to either a reduction or cessation of lung liquid secretion. The driving force for the net movement of water across the lung epithelium must be governed by the sum of all forces affecting liquid movement. Under normal conditions, a small hydrostatic pressure exists across the lungs because pressure within the lung lumen is 1 to 2 mm Hg greater than amniotic sac pressure. This small pressure gradient likely opposes the osmotic pressure resulting from the movement of chloride ions. The osmotic pressure promoting lung liquid secretion must exceed the opposing hydrostatic pressure for liquid to cross the epithelium into the lung lumen. Thus reductions or increases in the intraluminal pressure, resulting from alterations in lung liquid volume, would be expected to increase or reduce net lung liquid production rates by altering the magnitude of the opposing hydrostatic pressure (i.e., without directly affecting the ionic mechanism of lung liquid secretion).
Control of Fetal Lung Liquid Volume
For most of gestation, the fetal lung develops in an expanded state, and the volume of liquid within the future airways increases markedly over the last half of gestation. Over the last third of ovine gestation, lung liquid volume typically increases from 25 to 30 mL/kg to 45 to 50 mL/kg near term (145–150 days) ( Fig. 11.4 ). In contrast, the corresponding luminal volume of the air-filled lung (functional residual capacity (FRC)) is 15 to 20 mL/kg immediately after birth in newborn rabbits and 20 to 25 mL/kg in newborn lambs at 1 to 2 days of age. Thus, during late gestation, the fetal lung is hyperexpanded relative to the postnatal air-filled lung (see Fig. 11.4 ). The reduction in lung luminal volume (i.e., FRC) at birth is likely due to the formation at birth of an air–liquid interface, and hence surface tension, which constitutes the major part of the postnatal lung’s elastic recoil. Although the presence of surfactant greatly reduces surface tension, it does not eliminate it and, as a consequence, the lung tends to pull away from the chest wall after birth. This increased recoil after birth results in the formation of a subatmospheric pressure in both the intrapleural and the perialveolar interstitial tissue space which is not present in a fetus.
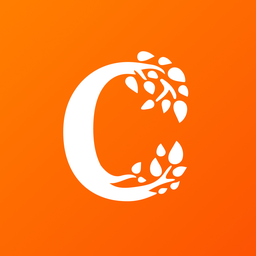
Full access? Get Clinical Tree
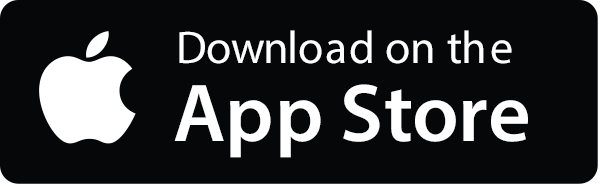
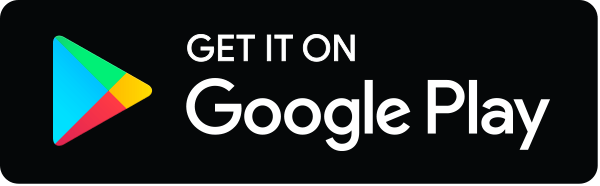