Life Support Systems
Julio Pérez Fontán
The past three decades have seen immense advances in the technologies used to replace the functions of failing organs or systems. During this time, mechanical ventilation has evolved from being used only as a last rite, when there was little hope of survival, to being a widely used and versatile technique that allows thousands of patients to recover from respiratory failure every year. Extracorporeal membrane oxygenation (ECMO), reintroduced into neonatal and pediatric intensive care medicine in the 1980s, is rapidly reaching similar status, as its indications and, perhaps more importantly, its limitations are better defined. More recently, external and implantable ventricular assist devices have undergone sufficient development to become viable alternatives for the continued support of patients with severe circulatory dysfunction, often as a bridge toward cardiac transplantation. While these technologies offer many possibilities, intensive care specialists must use them wisely by carefully selecting the patients who can benefit, by providing patients and families with realistic assessments of their potential, and by carefully evaluating the results to define better indications and to improve efficacy.
MECHANICAL VENTILATION
Today, any modern intensive care unit has at its disposal a wide array of ventilator models, many of them capable of effectively ventilating the lungs of adults as well as those of premature infants. Although these devices certainly make the task easier, their application to the treatment of infants and children requires more than a passing knowledge of respiratory physiology—it requires careful attention to monitoring the patient’s responses.
PRINCIPLES OF VENTILATORY SUPPORT
All commonly used methods of mechanical ventilation are based on the same principle: A mechanical device forces a gas mixture into the lungs during inspiration and then allows the passive recoil of lungs and chest wall to force gas out of the lungs during expiration. In most cases, the device uses a bellows or other type of pneumatic mechanism to inject a gas mixture into the airway lumen, either through a cannula inserted in the trachea or through a mask applied around the mouth and nose (positive-pressure ventilation). More rarely, the device lowers pressure around the patient’s chest and abdomen (negative-pressure ventilation), decreasing pleural and alveolar pressures relative to atmospheric pressure and drawing gas into the alveoli. Usually, a cannula must be placed in the trachea to prevent subatmospheric airway pressures from collapsing the pharynx and larynx during inspiration. Contrary to common misconception, positive- and negative-pressure ventilation apply similar strains to the lung tissue for a given level of lung inflation.3 Thus, it seems there would be no difference in the degree of lung injury caused by both methods, provided that tidal volume and functional residual capacity are kept equal. This consideration is, of course, limited by the practicality of its assumptions. Without entering a several-decade-long controversy on which method of ventilation is better,4 it seems clear that, by reducing rather than increasing pleural pressure relative to the atmosphere, negative-pressure ventilation may have a more beneficial effect on venous return to the heart than positive-pressure ventilation.5 However, the hemodynamic advantages do not make up for the impracticalities of negative-pressure ventilators, which include bulkiness, poor access to the patients, and inefficiency when high levels of support are needed.
The choice of an appropriate ventilatory strategy involves careful consideration of both the characteristics of the patient’s respiratory dysfunction and the ventilator’s capabilities. In general, the clinician must reconcile two fundamental and sometimes competing goals while designing such a strategy: (1) to maintain adequate gas exchange at the lowest possible inspired O2 concentration and (2) to minimize the accumulative effects of repeated stress and relaxation cycles on the lungs and airways.
STRATEGIES TO IMPROVE GAS EXCHANGE
The choice of optimal ventilatory pattern depends on the mechanical alterations produced by the disease in the lungs and airways, the homogeneous or heterogeneous distribution of these alterations, and the patient’s ability to contribute to the ventilatory effort. In practice, this choice materializes in a combination of several ventilatory settings, including ventilatory rate, tidal volume, inspiratory time, and inspiratory flow. These settings are organized into modes of ventilation, which are defined by the mechanisms that initiate, limit, and end (cycling) inspiration.
It is well established by both experimentation and clinical experience that no positive-pressure ventilator can duplicate the efficiency of the respiratory muscles.5 Even in individuals with healthy lungs, tidal volume, minute ventilation, and sometimes inspired O2 concentration must be raised above physiological levels to compensate for the increase in dead space and venous admixture that follows the institution of ventilatory support. The mechanisms of such increased requirements include the excessive distention of the conducting airways, the effacement of the vertical ventilation/perfusion gradients within the lungs, and the limitations imposed to movement and posture by the need to keep the endotracheal tube safely in place.6
ADMINISTRATION OF SUPPLEMENTAL O2
Increasing the concentration of O2 in the inspired gas is the most predictable and rapid method to increase arterial PO2 in patients with increased venous admixture caused by ventilation/perfusion inequality, decreased ventilation, and diffusion abnormalities. Unfortunately, O2 administration has no direct beneficial effect on the gas-exchanging mechanism itself. Moreover, the use of O2 can compound the gas-exchange derangements. The presence of high concentrations of O2 in poorly ventilated alveolar-capillary units can cause reabsorption atelectasis as O2 in the alveolar gas is removed by pulmonary capillaries without being replaced by N2. Oxygen is in itself highly toxic to the lung tissues, even though its continued administration upregulates local antioxidant responses.7 Based on these considerations, it is reasonable, when ventilatory support is started, to increase inspired O2 concentration as much as needed to correct hypoxemia. Thereafter, it is advisable to adopt other strategies that minimize the continued need for O2.
SELECTION OF TIDAL VOLUME AND VENTILATORY RATE
The product of ventilatory rate by the tidal volume determines the ventilator’s contribution to the total minute ventilation, the remainder of it being supplied by the patient’s spontaneous effort (if any). Tidal volume is usually preset by adjusting either the volume delivered into the ventilator’s circuit (volume-controlled ventilators) or, less frequently, the pressure generated at the airway opening (pressure-controlled ventilators). Some infant ventilators are time-cycled. When the safety pressure limit in these ventilators is set higher than the peak airway pressure, tidal volume is determined by the inspiratory flow circulating constantly through the circuit and by the duration of the inspiratory time (when the airway pressure exceeds the safety pressure, the ventilator behaves as if it were in the pressure-controlled mode). Many ventilators combine options for volume-, pressure-, and time-cycled control of tidal volume.
When instituting ventilatory support in a volume-controlled mode, the tidal volume generated by the ventilator is always greater than the actual tidal volume delivered into the lungs. The difference usually results from leaks and compression of the gas in the ventilator tubing. Leaks may be apparent as a discrepancy between the inhaled and exhaled volume. Although their exact magnitude is difficult to estimate, they can be quite large with the uncuffed endotracheal tubes commonly used in infants and small children and often vary with the position of the head. Because of gas leaks and compression (and frequent inaccuracies in volume measurement by the ventilator), the selection of an appropriate tidal volume should always be corroborated by the size of the chest wall excursions, independent of the information provided by the ventilator.
During pressure-controlled ventilation, tidal volume is determined by the magnitude and timing of the airway pressure increase during inspiration. In adjusting these two variables, it is important to remember that it is alveolar and not airway opening pressure that drives the change in lung volume. How airway and alveolar pressures relate to each other depends on the inspiratory flow and the combined resistance of the endotracheal tube and airways (Fig. 109-1). When inspiratory flow is constant (as is the case with volume-controlled modes of ventilation and with infant constant-flow time-cycled ventilators), airway opening pressure is greater than alveolar pressure throughout inspiration. Thus, the peak inspiratory pressure at the airway opening underestimates the peak alveolar pressure by a magnitude proportional to the inspiratory flow and the resistance of the tube and airways. When a decelerating inspiratory flow is applied (as is usually the case with pressure-controlled modes), airway opening and alveolar pressures equilibrate after gas flow ceases at the end of inspiration. Accordingly, peak airway opening and alveolar pressures are similar.
The choices of tidal volume and ventilatory rate are clearly interdependent. It is a good starting rule that their combination should mimic the patient’s own ventilatory pattern. Small tidal volumes at a relatively high ventilatory rate offer advantages in infants and children with severe restrictive disease. In contrast, slightly larger tidal volumes and a slower ventilatory rate are appropriate in patients with airway obstruction. Especially when the obstruction is intrathoracic, enough time must be allowed for expiration to be completed (Fig. 109-2). Otherwise, the subsequent inspiration interrupts expiratory flow, causing pressure in the alveoli to exceed that in the proximal airway. The resultant increase in lung volume is difficult to detect. If uncorrected, it may flatten the combined volume-pressure relationship of the lungs and chest wall, decrease tidal volume, and interfere with venous return to the heart.
POSITIVE END-EXPIRATORY PRESSURE
When lung volume decreases as a result of disease (eg, surfactant deficiency, pulmonary edema), alveoli lose their mechanical stability and collapse easily. Under such conditions, the number and volume of the alveoli that remain open is very sensitive to the volume of the preceding inflations (a property that physiologists call volume history dependence). This is why early ventilators were designed to provide periodic intermittent sighs or breaths larger than the prevalent tidal volume (a practice that has, incidentally, come back into vogue with some modifications and the new name of recruitment maneuvers). It soon became obvious that raising the functional residual capacity (FRC) of the lungs by increasing the airway pressure at the end of expiration was a much more effective alternative. Ostensibly, the objectives of positive end-expiratory pressure (PEEP) are to recruit collapsed alveoli and to prevent the closure of those that are open. When these goals are achieved, the ventilation/perfusion profile of the lungs improves and venous admixture decreases8 (see Fig. 102-5). As an added benefit, lung compliance and alveolar ventilation increase, improving the efficiency of mechanical ventilation while reducing the workload of the respiratory muscles in patients who also breathe spontaneously. However, when PEEP fails to recruit a predominance of collapsed or underventilated alveoli, well-ventilated alveoli become overdistended. Blood flow may then be forced through alveolar-capillary units with a low ventilation/perfusion ratio, overcoming their hypoxic vasoconstriction. As a result, venous admixture increases and lung compliance and alveolar ventilation decrease. The unpredictable nature of these changes emphasizes the importance of careful clinical assessment after changing ventilatory strategy.
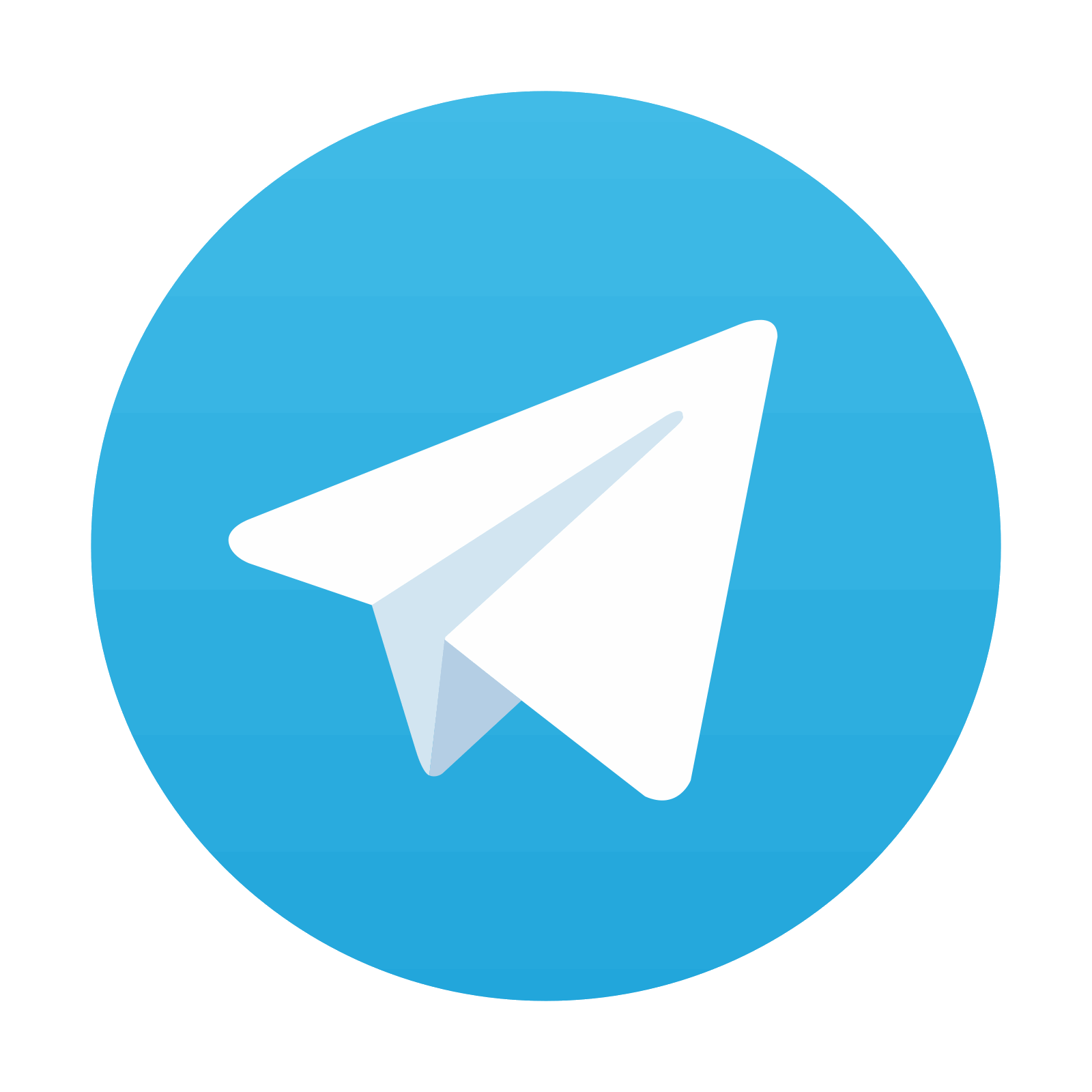
Stay updated, free articles. Join our Telegram channel
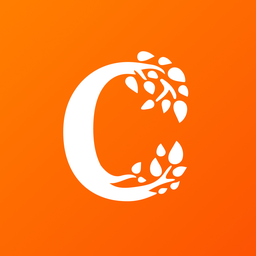
Full access? Get Clinical Tree
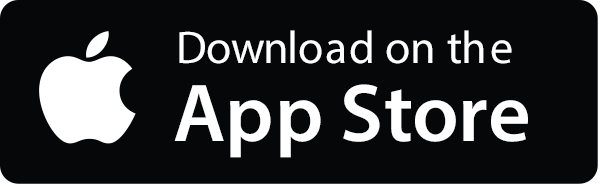
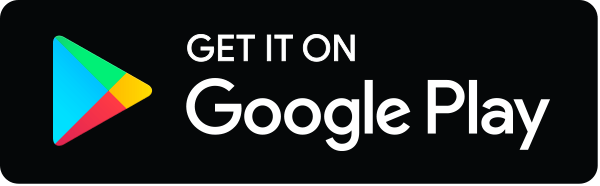