Invasive Hemodynamic and Oxygen Transport Monitoring During Pregnancy
Nan H. Troiano
Sreedhar Gaddipati
Invasive hemodynamic monitoring is a significant adjunct often used in the care of critically ill patients. Data obtained from this process may enhance the ability of critical care clinicians to assess a patient’s hemodynamic and oxygen transport status, formulate diagnoses, develop and implement appropriate plans of care, and evaluate patient responses over time.
Since its introduction into clinical practice over three decades ago, the flow-directed balloon-tipped pulmonary artery (PA) catheter has played an integral role in the management of critically ill patients in a number of specialties, including obstetrics.1,2,3,4,5 Intra-arterial catheters and PA catheters represent the two fundamental modalities for invasive hemodynamic assessment. This chapter reviews the fundamental principles related to invasive central hemodynamic and oxygen transport monitoring during pregnancy. Clinical case excerpts are presented to reinforce select principles regarding interpretation of data. More thorough discussion of clinical applications of these principles as they relate to the care of patients with specific obstetric complications is presented elsewhere in this text.
Cardiac Anatomy and Physiology
Delivery of adequate oxygen, nutrients, and other vital substances in the blood to tissues is necessary for cellular function that sustains life. The role of the cardiovascular system is to pump these substances through the vascular bed by contraction of the heart. Normal cardiac anatomy is depicted in Figure 4-1.
Atria are low-pressure chambers that serve as reservoirs of blood for the ventricles. The right atrium receives venous blood via the superior and inferior vena cavae and coronary sinus from the systemic bed. The left atrium receives oxygenated blood returning to the heart from the pulmonary bed via the four pulmonary veins. Approximately 70 to 80 percent of blood flows passively from the atria to the ventricles during early ventricular diastole, known as protodiastole. During the later phase, the atria contract and pump an additional 20 to 30 percent of blood into the ventricles. Loss of atrial systole, also referred to as atrial kick, in an otherwise normal heart usually has only minimal effect. However, in a patient with impaired left ventricular filling, left atrial systole is very important and may account for more than 50 percent of left ventricular filling.
The ventricles provide the force necessary to circulate blood through the lungs and the rest of the body. The right ventricle pumps deoxygenated blood into the pulmonary circulation via the pulmonary artery. The left ventricle, much thicker than the right, pumps oxygenated blood into the systemic circulation via the aorta. The left side of the heart functions as a higher pressure system than the right side. Under normal circumstances, the right ventricle ejects approximately 50 to 60 percent of its end-diastolic volume with each cardiac contraction, whereas the left ventricle ejects 60 to 70 percent.
Atrioventricular valves consist of the tricuspid valve on the right and mitral valve on the left. During diastole, valve leaflets open, which allows unidirectional blood flow into the respective ventricle. As ventricular pressure increases during systole, valve leaflets close, which prevents retrograde flow. Semilunar valves consist of the pulmonic valve on the right and aortic valve on the left. Open during ventricular systole, each of these valves allows unidirectional blood flow to the respective arterial outflow tract. Following systole, as arterial pressures increase, each valve closes to prevent retrograde flow during diastole.
Determinants of Cardiac Output
Cardiac output is the amount of blood ejected from the heart per unit of time. It is determined by four variables: preload, afterload, contractility, and heart rate.
Preload
Preload refers to the tension or load on a muscle as it begins to contract. In the context of the cardiovascular system, preload is the length of the ventricular muscle fiber at end-diastole. The principal factor that determines muscle fiber length is the volume of blood in the ventricles at the point of maximal filling. Therefore, ventricular end-diastolic volume (EDV) is used as a reflection of preload for the intact heart. The pressure-volume curves in Figure 4-2 depict the influence of preload on the mechanical performance of the left ventricle during diastole (lower curves) and systole (upper curves). The solid lines on the curves represent the normal pressure-volume relationships during diastole and systole. Note that the uppermost curve in the figure has a rapid ascent, which indicates that small changes in EDV are associated with large changes in systolic pressure. For this reason, the most effective measure for preserving adequate cardiac output is to maintain an adequate EDV.
![]() Figure 4-2 Pressure–volume curves for the intact ventricle. Solid lines represent normal pressure-volume relationships. Dotted lines represent abnormal pressure-volume relationships. |
The intrinsic ability of the heart to adapt to alterations in the volume of blood returning to the heart is commonly known as the Frank-Starling phenomenon. The graph that is most often used to depict this relationship is known as a ventricular function curve (Fig. 4-3). Within certain physiologic limits, the more the ventricles are filled with blood during diastole, the greater the quantity of blood that will be ejected during systole.
Conversely, when the amount of blood returning to the ventricles is diminished, cardiac output may be impaired. For example, this may be evident in the patient with significant blood loss or hypotension who has decreased circulating blood volume or venous return to the heart.
Conversely, when the amount of blood returning to the ventricles is diminished, cardiac output may be impaired. For example, this may be evident in the patient with significant blood loss or hypotension who has decreased circulating blood volume or venous return to the heart.
The stretch imposed on cardiac muscle is determined not only by the volume of blood in the ventricular chambers but also by the tendency of the ventricular wall to distend or stretch at a given volume within the chamber. This property is described as the compliance of the ventricle. Compliance is determined by the relationship between changes in end-diastolic pressure (EDP) and end-diastolic volume. The lower curve in Figure 4-3 illustrates this concept. As the ventricle becomes less compliant (e.g., in ventricular hypertrophy), there is less change in diastolic volume relative to the change in diastolic pressure. Early in the process, the EDV remains normal, but the EDP increases above normal. As the compliance decreases further, the increase in EDP eventually reduces venous return to the heart, thereby causing a reduction in EDV.6
Afterload
Afterload is the resistance or load that opposes ventricular ejection of blood during systole. The right ventricle pumps against pressure or resistance in the pulmonary vasculature, whereas the left ventricle pumps against the higher pressure or resistance in the systemic circulation. In contrast to preload, afterload and cardiac output have an inverse relationship. Within certain physiologic limits, the lower the afterload or resistance applied against the ventricles during systole, the greater the cardiac output. Conversely, patients with clinical conditions such as pulmonary or systemic hypertension, in which pressure in the pulmonary or systemic vessels respectively is increased, are at risk for decreased cardiac output. Forces that contribute to ventricular wall tension or afterload are listed in Box 4-1.
Box 4-1. Forces That Contribute to Ventricular Tension or Afterload
Transmural wall tension
Systolic pressure
Pleural pressure
Outflow impedance
Chamber radius
End-diastolic volume
Vascular compliance
Vascular resistance
Because afterload is a transmural force, it is influenced by the pleural pressures at the outer surface of the heart. Negative pleural pressures increase transmural pressure and increase ventricular afterload, whereas positive pleural pressures have the opposite effect. Negative pressures surrounding the heart may impede ventricular emptying by opposing the inward displacement of the ventricular wall during systole.7 This action is responsible for the decrease in systolic blood pressure that occurs during the inspiratory phase of spontaneous breathing. Positive pleural pressures can promote ventricular emptying by facilitating the inward displacement of the ventricular wall during systole.
Contractility
Contractility, also known as the inotropic state of the heart, is the intrinsic ability of the heart muscle to shorten or develop tension independent of variations in preload and afterload. Not easily measured in clinical situations, contractility may nonetheless be inferred by a change in cardiac output when afterload and preload remain constant. Under conditions of altered catecholamine production or following administration of medications that alter inotropic response, the Frank–Starling curve shifts upward with a higher cardiac output for a given filling pressure. Conversely, with decreased contractility, the heart pumps less well at a given filling pressure. Thus, patients with compromised heart function at higher pressures under normal conditions have less reserve and are more prone to heart failure when stressed.
Heart Rate
Heart rate affects the strength of myocardial contraction and thus cardiac output. For example, at faster rates, the force of contraction is strong. At slower rates, the force is weaker. This intrinsic property of the heart muscle may be attributable to rate-driven variations in sarcoplasmic calcium concentration. It should be noted that an increased heart rate also results in decreased diastolic filling time and may thereby decrease preload. If continued over a period of time, this may lead to decreased cardiac output. In addition, a pause between heart contractions results in increased force of the next contraction, also known as rest potentiation, which may increase cardiac output. For these reasons, rate-related changes in the force of contraction, preload, and cardiac output interact in a complex manner.
Oxygen Transport Physiology
The ability of cells to function normally depends on a continuous supply of adequate oxygen and nutrients. When oxygen transport is impaired, the patient is at
increased risk for end-organ dysfunction or failure. Thus, evaluation of oxygen transport is essential in the care of obstetric patients with significant complications or who are critically ill. Key concepts related to oxygen transport include oxygen content, affinity, delivery, and consumption.
increased risk for end-organ dysfunction or failure. Thus, evaluation of oxygen transport is essential in the care of obstetric patients with significant complications or who are critically ill. Key concepts related to oxygen transport include oxygen content, affinity, delivery, and consumption.
Oxygen Content
Oxygen is transported to the tissues in two ways: dissolved under pressure in plasma and bound to hemoglobin in red blood cells. The oxygen dissolved in plasma makes up approximately 1 to 2 percent of the total oxygen content, whereas oxygen bound to hemoglobin makes up the remaining 98 to 99 percent.
The role of hemoglobin in the transport of oxygen is significant. Hemoglobin is composed of four subunits, each consisting of a protein chain with a heme group attached to a histidine residue. One molecule of oxygen is loosely bound with one of the six coordinate valencies of each of the heme iron atoms. Thus, each molecule of hemoglobin is associated with four molecules of oxygen. The kinetics of the association are such that all of the molecules of oxygen bind at the same rate. When a hemoglobin molecule is combined with oxygen, it is called oxyhemoglobin. The saturation of hemoglobin with oxygen is the ratio of oxyhemoglobin to the total amount of hemoglobin capable of transporting oxygen (Fig. 4-4).
Although it represents only a small fraction of total oxygen content, oxygen dissolved in plasma plays a crucial role. The ability of oxygen to combine with hemoglobin in the lungs, later to be released at the tissue level, is affected by oxygen in the plasma. This reversible binding of oxygen to hemoglobin is an important concept in oxygen transport and allows for the loading of oxygen in the lungs and unloading at the tissue level.
Oxygen Affinity
Affinity refers to the ability of oxygen to bind to hemoglobin. Both the uptake and the release of oxygen from hemoglobin molecules are represented visually by the oxyhemoglobin dissociation curve, depicted in Figure 4-5.
The curve portrays the relationship between the partial pressure of oxygen (PaO2) in arterial blood and the saturation of hemoglobin (SaO2). The sigmoid shape of the normal curve is the result of:
the increased affinity of hemoglobin for oxygen as more oxygen molecules combine with it, despite large alveolar PO2 changes (the flat, upper portion of the curve) and
the rapid unloading of oxygen from hemoglobin, with small changes in PaO2 (the steep, lower portion of the curve).
The position of the curve is defined more precisely by a reference point known as the P50. This point represents the PaO2 at which hemoglobin is 50 percent saturated. Under normal conditions in nonpregnant people, the P50 is 26.3 mmHg (see Fig. 4-5).
The P50 is not fixed in those who are critically ill. If the affinity and P50 change, the oxyhemoglobin dissociation curve shifts to the right or left. Conditions known to change oxygen affinity are described in Table 4-1. Decreased oxygen affinity, resulting in a right shift of the
oxyhemoglobin dissociation curve, means that at any given PaO2, there is decreased saturation. Thus, oxygen is released more readily to tissues. Conversely, increased oxygen affinity, resulting in a left shift of the curve, means that at any given PaO2, there is increased saturation. This results in oxygen binding more tightly to hemoglobin.
oxyhemoglobin dissociation curve, means that at any given PaO2, there is decreased saturation. Thus, oxygen is released more readily to tissues. Conversely, increased oxygen affinity, resulting in a left shift of the curve, means that at any given PaO2, there is increased saturation. This results in oxygen binding more tightly to hemoglobin.
Table 4.1 Conditions Known to Change Oxygen Affinity to Hemoglobin | ||||||||||
---|---|---|---|---|---|---|---|---|---|---|
|
It should be noted that pregnancy also affects the position of the oxyhemoglobin curve. The maternal curve normally shifts to the right, whereby oxygen is released more quickly from hemoglobin. The fetal curve normally shifts to the left, resulting in increased affinity of oxygen for hemoglobin. This concept is depicted in Figure 4-6.
Oxygen Delivery
Oxygen delivery (DO2) is the amount of oxygen delivered to the tissues per unit of time. The delivery of oxygen from the lungs to the tissues depends on cardiac output as well as on the content of oxygen in arterial blood (CaO2). It should be recalled that cardiac output is dependent on preload, afterload, contractility, and heart rate. Arterial oxygen content is determined by the arterial concentration of hemoglobin, the arterial saturation of hemoglobin (SaO2), and the amount of oxygen dissolved under pressure in plasma within the arteries (PaO2).
Factors that increase cardiac output (e.g., uterine contractions, increased metabolism, and certain medications) also increase DO2. In addition, factors that increase total oxygen content (e.g., transfusion of packed red blood cells) increase DO2. Such conditions may include hypovolemia, hypoxemia, anemia, and administration of certain medications. When oxygen supply is threatened, the body attempts to compensate in order to maintain delivery to the tissues by first increasing cardiac output. It should be noted that cardiac output may increase severalfold above normal in healthy people under stressful circumstances.
Oxygen Consumption
Oxygen consumption (VO2) refers to the amount of oxygen consumed by the tissues each minute. At rest, VO2 is approximately 25 percent of the total oxygen available. Oxygen consumption is increased by numerous conditions as well as by interventions, therapeutic procedures, and various other stressors. It should be noted that VO2 also increases significantly during normal pregnancy.
The normal relationship between DO2 and VO2 is such that sufficient reserve exists to maintain VO2 independent of DO2 over a wide range of delivery values. However, situations may develop whereby prolonged decreased DO2, below a critical threshold, eventually results in a linear fall in VO2. This is known as delivery dependent VO2 because the amount of oxygen consumed is limited by the amount of oxygen delivered. In such circumstances, VO2 does not become independent of DO2 except at very high levels of oxygen delivery, if at all. This pathologic response is related to the fixed oxygen-extraction ratio present in some critically ill patients.
Central Hemodynamic Assessment
Invasive hemodynamic monitoring may be accomplished via use of a central venous or pulmonary artery catheter. The description, assessment capabilities, indications, and associated complications for each follow.
Central Venous Pressure Catheter
The central venous pressure (CVP) catheter is a single- or multiple-lumen catheter advanced through a peripheral or central vein until the tip is in the proximal superior vena cava (Fig. 4-7). Central venous access is most commonly obtained via either the internal jugular or subclavian vein. The internal jugular vein is preferred during pregnancy because of the increased risk of pneumothorax with subclavian placement attempts during pregnancy.
Use of this catheter permits evaluation of right preload (expressed in mmHg as CVP), as well as access for administration of fluid or medications. The primary limitation with respect to central hemodynamic assessment is that right ventricular function may not accurately reflect left ventricular function. Thus, clinical use of the CVP may be misleading and possibly deleterious in certain clinical situations. In addition, a CVP catheter does not permit evaluation of data regarding other determinants of cardiac output or oxygen transport parameters. For these reasons, use of a CVP catheter for assessment of hemodynamic function in a critically ill obstetric patient is seldom, if ever, indicated. However, CVP catheters may be utilized for reasons other than assessment of hemodynamic function. Box 4-2 lists potential indications for use of a CVP catheter.8
Complications of central venous access that can occur during insertion and monitoring include pneumothorax, venous air embolism, inadvertent arterial puncture, and infection. Although pneumothorax has been reported in 1 to 5 percent of a general patient population undergoing central venous catheterization, Clark and associates observed no pneumothorax when the internal jugular approach was used in a compilation of obstetric and gynecologic patients.2 Strategies to reduce the risk of central line–associated bloodstream infection (CLABSI) are presented later in this chapter.
Box 4-2. Potential Indications for Use of a Central Venous Pressure Catheter
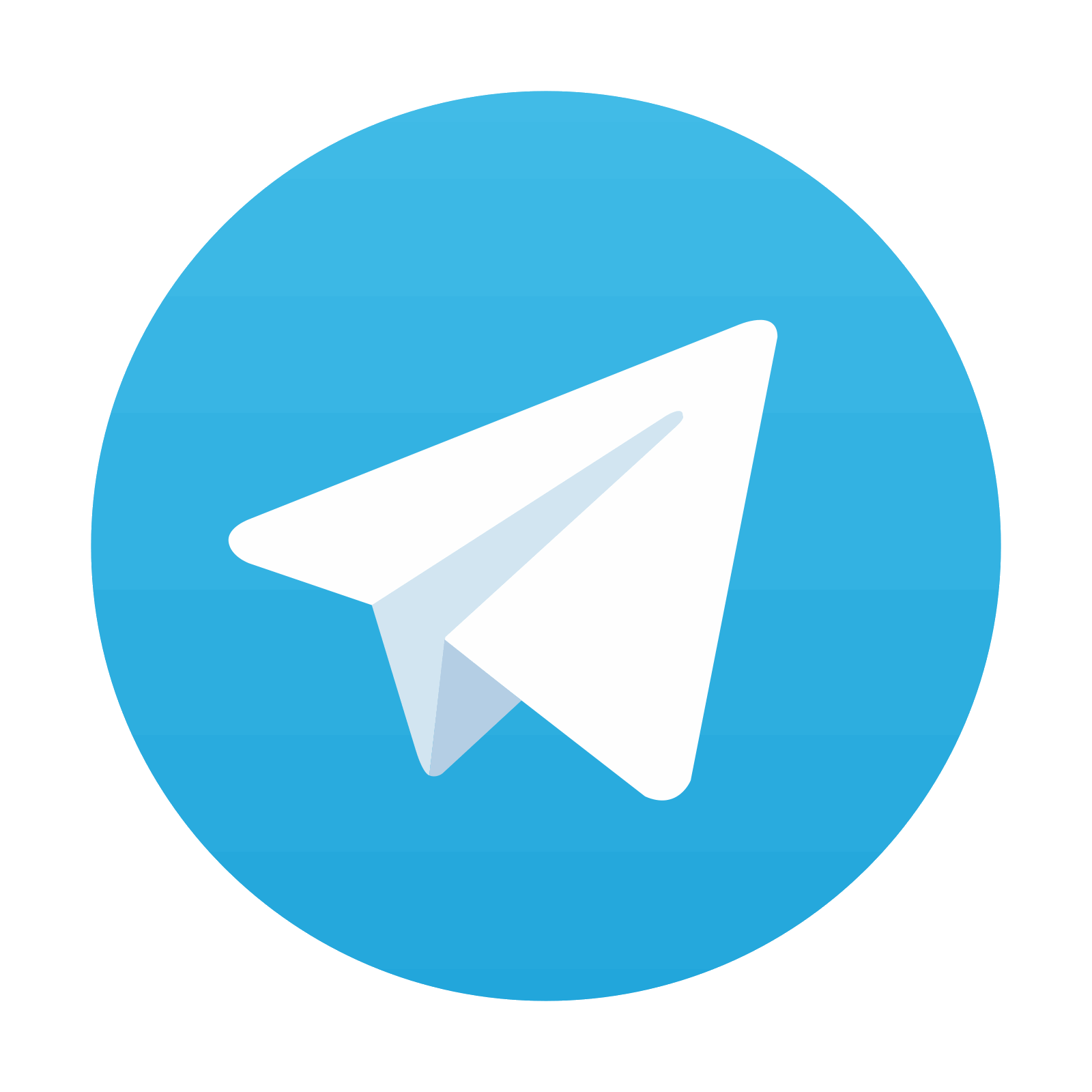
Stay updated, free articles. Join our Telegram channel
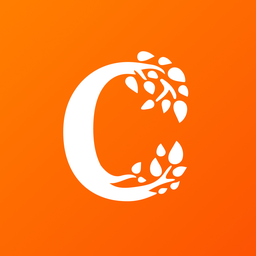
Full access? Get Clinical Tree
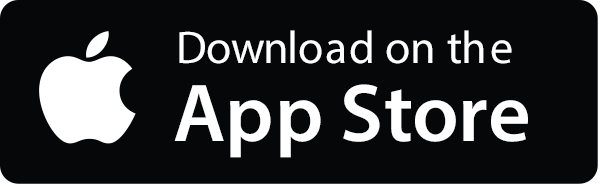
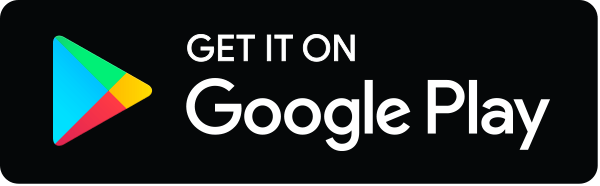
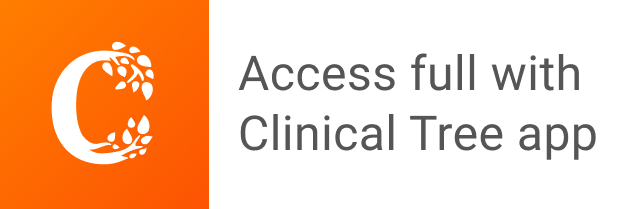