Introduction
Sleep is a vital process, characterized by decreased consciousness with inhibited motor activity and rapid reversibility. Respiratory physiology during sleep undergoes a multitude of maturational changes during childhood, especially in infancy.
Overview of infant breathing during sleep
Since infants spend most of their time sleeping, understanding normal patterns and maturation of breathing during sleep is crucial. It is equally important to recognize the difference in breathing physiology between sleep and wakefulness as well as between different sleep stages, namely rapid eye movement (REM) and non-REM (NREM) sleep. For example, a decrease in functional residual capacity (FRC) in sleep is particularly marked in REM. In REM sleep (versus NREM), respiratory rate (RR) is higher and more variable. Ventilatory responses to hypoxia and hypercapnia are attenuated in sleep, particularly in REM (versus NREM) sleep. ,
Maturation of sleep architecture takes place over several years of early childhood. In newborn infants, sleep stages can be categorized into REM versus NREM sleep, which are equivalent to “active” and “quiet” sleep, respectively, which represent a more organized sleep architecture. During quiet sleep, breathing is regular and manifests episodic sighs that are followed by oscillatory breathing. The response to sigh or recovery of tidal volume to presigh values is stable but slow in the first few days of life in newborns. During the period between 4 to 8 days and 3 to 4 months of life, the oscillatory period decreases, and respiration becomes potentially more stable. By 3 to 4 months, the response becomes more stable with a rapid response time.
In active/REM sleep, breathing is irregular in terms of RR and tidal volume, with a variable oscillatory pattern. In newborn infants, pauses in breathing of up to 10 seconds are common, particularly in active sleep and the frequency of this decreases with age. During active sleep (REM), muscle tone is diminished. Low intercostal muscle tone then leads to paradoxical movement of rib cage that is partially compensated by increased diaphragmatic work of breathing but at the expense of potential diaphragmatic fatigue in REM sleep. As a result, so-called “expiratory braking” can be lost. In the immediate newborn period, the first breaths tend to be deeper and longer than subsequent breaths and are characterized by a short deep inspiration followed by a prolonged expiratory phase. This expiratory braking helps to develop and maintain FRC in the newborn period when the chest wall is very compliant. These series of breathing changes make REM sleep the most vulnerable stage for respiratory disturbances. Moreover, diminished muscle tone in upper airway muscles makes infants susceptible to airway obstruction in REM sleep, especially in infants with anatomical susceptibility such as macroglossia and micrognathia.
NREM/quiet sleep is characterized by a generally regular breathing pattern, punctuated by intermittent sigh breaths. During NREM sleep, there is a decrease in RR and depth compared with the awake state, resulting in decreased minute ventilation. REM sleep, on the other hand, is characterized by more irregular breathing, in both RR and tidal volume. During REM sleep, there is inhibition of skeletal muscle tone affecting the intercostal muscles and leading to thoracoabdominal asynchrony and increased diaphragmatic work of breathing. This paradoxical incursion of the rib cage with inspiration is often seen with younger postnatal age and can be expected up to 3 years of age.
Normal values for hemoglobin oxygen saturation (SpO 2 ) reach at least 80% within 10 minutes of birth in term and healthy preterm infants without oxygen supplementation. Arterial partial pressure of oxygen (PaO 2 ) values of 50 to 80 mmHg are sufficient to meet the metabolic demand in the newborn partly owing to the high proportion of fetal hemoglobin (HbF). Normative data for preterm infant are scarce. In one study that included a group of preterm infants during the first days of life, oxyhemoglobin desaturation episodes (SpO 2 <80%) were found on 10 of 55 recordings. Baseline values during regular breathing ranged from 90% to 100%. Most oxyhemoglobin desaturation events are related to apneic episodes. The number of episodes diminishes with time. Lower baseline O 2 levels at first week of postnatal age than at 1 month may be due to age-related differences in intrapulmonary and intracardiac shunting. Decrease in minute ventilation, combined with reduced intercostal muscle tone and supine positioning, all contribute to reduced oxygenation.
In full-term infants, the mean SpO 2 in REM sleep is approximately 4% less than that in NREM sleep at 1 week of age. This is due to low O 2 tensions, high incidence of apnea, high metabolic consumption, and a decrease in the O 2 stores in REM sleep. End-tidal CO 2 (ETCO 2 ) monitoring is limited at this age due to technical issues related to elevated RR that preclude a good ETCO 2 signal plateau. Schafer et al. found no changes in transcutaneous CO 2 measurements (tcCO 2 ) with postnatal age in newborns but the mean and maximum CO 2 decreases with time during the first 18 months of life. The lower values of tcCO 2 in REM than NREM may be due to paradoxical sleep coupled with increased O 2 consumption.
Chest wall and lung mechanics
Growth accompanies changes in chest wall geometry. Hence, the chest wall undergoes several maturational changes in the first few years of life, summarized below.
There is a striking difference in the cross-sectional shape of the infant rib cage compared to older children and adults. The chest wall shape of an infant almost resembles a circle, and with age, becomes more ovoid. The adult chest wall shape is achieved by roughly 3 to 5 years of age.
The positioning of the ribs affects chest expansion in infancy. The relatively horizontal position of the ribs results in inefficient expansion of the chest wall. Then, when the hemidiaphragms contract in the setting of horizontal ribs, there is worsening distortion of the compliant infant chest wall. In older children and adults, on the other hand, ribs are downward sloping which allows for greater chest expansion by lifting the anterior ribs.
In both the prenatal and postnatal periods, the diaphragm undergoes developmental changes in terms of muscle fiber composition and neuronal properties, impacting its contractility and endurance. In early infancy, the left hemidiaphragm dome is positioned around vertebral level 8. However, this hemidiaphragm descends to around level T11 as a child approaches 3 years of age. Throughout life, the right hemidiaphragm is typically positioned approximately half a vertebral level higher.
Changes in compliance
Compliance, defined as the change in volume (L) per unit change in pressure (cm H 2 O), can be measured for the lungs, chest wall, and total respiratory system. The lungs and chest wall have distinct, nonlinear relationships between volume and pressure. Compliance can be further broken down into static compliance and dynamic compliance. Static compliance can be ascertained at a fixed volume, while dynamic compliance is measured with normal breathing.
The lungs become inflated with inspiration and then recoil inward due to their elastic properties. Conversely, the chest wall exerts outward recoil. The FRC denotes the point at which the inward recoil of the lungs is equal to the outward recoil of the chest wall, and it is dependent on the passive elastic properties of the respiratory system. Lung compliance does not significantly change with age, even when adjusted for body size. However, the highly compliant chest wall of the neonate leads to a lower passive resting lung volume compared to older children and adults.
In infancy and in later stages of life, FRC is reduced during sleep relative to the awake state; this is more pronounced during REM sleep. However, the compliance of the chest wall of newborns and infants is easily compromised when the diaphragm is contracted or when the intercostal muscles are inhibited, as occurs during REM sleep. In REM sleep, as previously mentioned, phasic inspiratory diaphragm contractions coupled with the decreased intercostal muscle tone cause paradoxical breathing; the rib cage moves inward rather than outward. In full-term newborns, rib cage distortion occurs nearly 100% of REM sleep periods.
Changes in control of breathing
In newborns, peripheral chemoreceptors play a central role in initiating the ventilatory, cardiovascular, and arousal responses to hypoxia or asphyxia. Dramatic resetting of the peripheral chemoreceptors occurs in early postnatal life especially during the first 2 weeks of life.
The hypoxic ventilatory response (HVR) refers to change in minute ventilation in response to hypoxia. Newborns exhibit biphasic HVR; transient increase in ventilation, followed by a decrease to or below baseline levels. Interestingly, however, in infants born at 32 to 37 weeks, hyperventilation response to hypoxia does not occur in a cool environment. This biphasic response is thought to be due to central depression (versus peripheral chemoreceptors), decrease in metabolic rate, and changes in lung mechanics. Premature infants may show a biphasic HVR response up to 25 days after birth.
The hypercapnic ventilatory response (HCVR) is the ventilatory response to CO 2 , also expressed as changes in minute ventilation (L/minute) by mmHg of CO 2 (PCO 2 ). Conceptually, it measures CO 2 sensitivity. HCVR is influenced by age, sex, weight, body surface area, vital capacity, and genetic polymorphism. The HCVR corrected for weight decreases with age. HCVR is reduced in premature infants mostly due to a limited RR response to increased CO 2 despite a more substantial tidal volume response to CO 2 . The HCRV increases as the infants mature. For the HCVR measurement, controlled setting such as rebreathing or steady state is needed.
HCRV is reduced in premature infants of less than 33 weeks gestational age. HCRV increases as 40 weeks postconceptional age is approached. In one study, HCRVs (unadjusted for body size) were 0.044 L/min/mmHg for <33 weeks, 0.174 for 33 to 36 weeks, and 0.292 for 37 to 40 weeks infants. In full term infants, the HCRV mostly increases over the first 2 days but continues to mature over 8 weeks of life. In the study by Søvik et al., 26 healthy term infants were assessed for ventilatory response to hypercapnia, hypoxia, and hypercapnic hypoxia in a randomized sequence. Although response magnitude to hypercapnia did not change, response rate to hypercapnia increased over 8-week period, suggesting ventilatory response continues to mature between postnatal between birth and 8 weeks in term infants.
Obtaining HVR and HCVR during sleep is more complicated during sleep than during wakefulness even more so in infants as they do not have the developmental ability to express distress and cooperate with the measurements. Therefore, most available sleep data come from neonatal animal model.
Ventilatory drives are important in the maintenance of ventilation during sleep but both HVR and the HCRV decrease during sleep. Reduction in HVR is more pronounced in REM versus NREM sleep in adults. This may explain the REM-associated hypoxemia in both healthy infants and in those with cardiopulmonary disease. Minimal data exist on the effect of sleep stage on the HVR in infants due to the difficult set-up that such a test entails in noncooperative subjects. One study showed that HVR is more variable during REM than NREM sleep when assessed at 2 to 5 weeks, 2 to 3 and 5 to 6 months.
HCRV markedly decreases in sleep in infants. The degree of HCRV decrease appears to be similar between REM and NREM sleep. In one study where the effect of CO 2 on the ventilatory pattern of 18 normal infants was assessed during sleep through the age of 4 months, the percent increase from base line in instantaneous minute ventilation during REM sleep was similar to that during NREM sleep.
There has been a suggestion that sleep position can affect ventilatory response. In prematurely born infants, some studies have shown that respiratory control can be more vulnerable in supine sleep. In one study including premature infants (mean post conceptional age 35 weeks), the supine position was associated with a higher RR and lower SpO 2 than the prone position. HCVR was lower in the supine than in the prone position. Rib cage contribution to ventilation in the supine was less than in the prone position. Ventilatory and arousal responses to mild asphyxia (hypercapnia/hypoxia) did not differ between supine and prone position in healthy infants at newborn but the response was diminished in prone position at 3 months of age, particularly in active sleep. In contrast, some studies reported opposite findings that propose position is associated with impaired ventilatory response to CO 2 challenge in preterm infant asserting that such compromise in prone position may make preterm infant vulnerable to the SIDS. In a study that included preterm infants with symptomatic apnea and bradycardia (gestational age of 26.9 weeks), no difference in the incidence of clinically significant apnea, bradycardia, or desaturation between supine and prone positions was seen. Thus, there is an ongoing debate about which sleep position is more disadvantageous to breathing and whether sleep position-related breathing instability contributes to the SIDS.
Central and obstructive apneas
Central pauses, or central apneas, in the form of periodic breathing are commonly observed in newborns. With increasing postnatal age, the frequency of central apnea decreases due to a more mature respiratory drive, increased FRC, and a less compliant chest wall. , While longer central apneas can occur in term infants in the first month or so of life, they are more frequently seen in preterm babies. Periodic breathing, defined by 3 central pauses lasting at least 3 seconds in a period of respiration of 20 seconds or less, is commonly observed in the first few months of life but tends to decline in frequency after about 4 to 5 months of age. Periodic breathing is also more pronounced in warm settings. The frequency, mean, and maximum durations of apnea were correlated with the body heat loss in preterm neonate. However, in a case control setting, one study found that infants who later died of SIDS had experienced significantly more frequent episodes of obstructive and mixed sleep apnea than control group preceding SIDS. However, whether apnea of infancy is simply a marker (versus causal) of prematurity rather than the causal factor of SIDS is unclear.
Infants have both anatomical and physiological properties subjecting them to tendency of airway obstruction and gas exchange abnormalities. The newborn trachea and larynx are relatively superiorly positioned making epiglottis and soft palate proximal to each other. The infant mandible is nearly horizontal at birth resulting in a propensity toward posterior displacement. Adenotonsillar hypertrophy may become an important contributor to OSA in infants after 6 months of age. Besides these anatomical features, high chest wall compliance, ventilation-perfusion mismatching, and ventilatory control instability all contribute to the risk of OSA in infants. Congenital abnormalities of the airway, beyond the scope of this chapter, such as laryngomalacia, will have further effects on airway patency.
Conclusion
Both sleep and breathing are physiological processes that undergo progressive changes during infancy. Major changes for both sleep and breathing occur during the first months after birth. Breathing and ventilatory responses become more stable in the first months of life, and REM sleep represents the most vulnerable stage for respiratory instability. Respiratory events, including apneas, are more common in infants and decrease in healthy children.
References
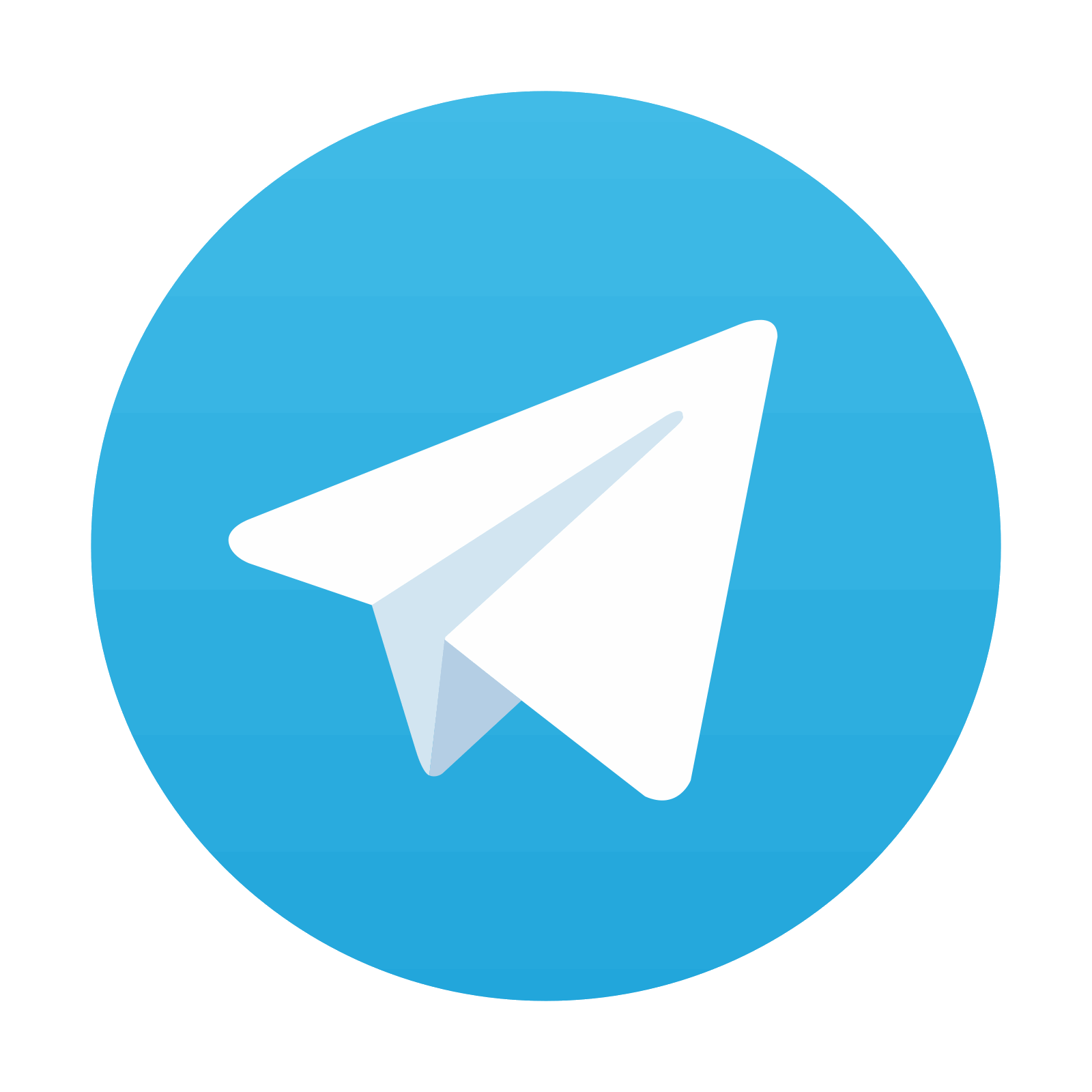
Stay updated, free articles. Join our Telegram channel
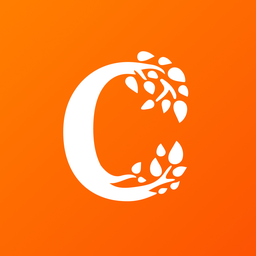
Full access? Get Clinical Tree
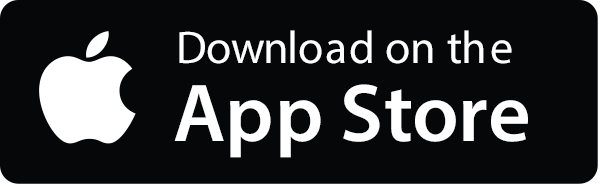
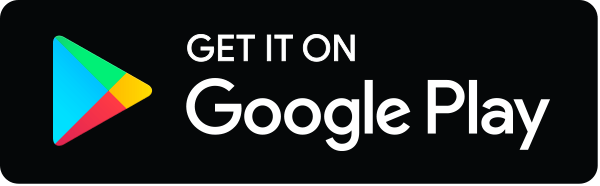