FIGURE 5-1 Gonadotropin control of the ovarian and endometrial cycles. The ovarian-endometrial cycle has been structured as a 28-day cycle. The follicular phase (days 1 to 14) is characterized by rising estrogen levels, endometrial thickening, and selection of the dominant “ovulatory” follicle. During the luteal phase (days 14 to 21), the corpus luteum (CL) produces estrogen and progesterone, which prepare the endometrium for implantation. If implantation occurs, the developing blastocyst begins to produce human chorionic gonadotropin (hCG) and rescues the corpus luteum, thus maintaining progesterone production. FSH = follicle-stimulating hormone; LH = luteinizing hormone.
The Ovarian Cycle
Follicular or Preovulatory Ovarian Phase
The human ovary contains 2 million oocytes at birth, and approximately 400,000 follicles are present at puberty onset (Baker, 1963). The remaining follicles are depleted at a rate of approximately 1000 follicles per month until age 35, when this rate accelerates (Faddy, 1992). Only 400 follicles are normally released during female reproductive life. Therefore, more than 99.9 percent of follicles undergo atresia through a process of cell death termed apoptosis (Gougeon, 1996; Kaipia, 1997).
Follicular development consists of several stages, which include the gonadotropin-independent recruitment of primordial follicles from the resting pool and their growth to the antral stage. This appears to be controlled by locally produced growth factors. Two members of the transforming growth factor-β family—growth differentiation factor 9 (GDF9) and bone morphogenetic protein 15 (BMP-15)—regulate granulosa cell proliferation and differentiation as primary follicles grow (Trombly, 2009; Yan, 2001). They also stabilize and expand the cumulus oocyte complex in the oviduct (Hreinsson, 2002). These factors are produced by oocytes, suggesting that the early steps in follicular development are, in part, oocyte controlled. As antral follicles develop, surrounding stromal cells are recruited, by a yet-to-be-defined mechanism, to become thecal cells.
Although not required for early follicular maturation, follicle-stimulating hormone (FSH) is required for further development of large antral follicles (Hillier, 2001). During each ovarian cycle, a group of antral follicles, known as a cohort, begins a phase of semisynchronous growth based on their maturation state during the FSH rise in the late luteal phase of the previous cycle. This FSH rise leading to follicle development is called the selection window of the ovarian cycle (Macklon, 2001). Only the follicles progressing to this stage develop the capacity to produce estrogen.
During the follicular phase, estrogen levels rise in parallel to growth of a dominant follicle and to the increase in its number of granulosa cells (see Fig. 5-1). These cells are the exclusive site of FSH receptor expression. The rise of circulating FSH levels during the late luteal phase of the previous cycle stimulates an increase in FSH receptors and subsequently, the ability of cytochrome P450 aromatase within granulosa cells to convert androstenedione into estradiol. The requirement for thecal cells, which respond to luteinizing hormone (LH), and granulosa cells, which respond to FSH, represents the two-gonadotropin, two-cell hypothesis for estrogen biosynthesis (Short, 1962). As shown in Figure 5-2, FSH induces aromatase and expansion of the antrum of growing follicles. The follicle within the cohort that is most responsive to FSH is likely to be the first to produce estradiol and initiate expression of LH receptors.
FIGURE 5-2 The two-cell, two-gonadotropin principle of ovarian steroid hormone production. During the follicular phase (left panel), luteinizing hormone (LH) controls theca cell production of androstenedione, which diffuses into the adjacent granulosa cells and acts as precursor for estradiol biosynthesis. The granulosa cell capacity to convert androstenedione to estradiol is controlled by follicle-stimulating hormone (FSH). After ovulation (right panel), the corpus luteum forms and both theca-lutein and granulosa-lutein cells respond to LH. The theca-lutein cells continue to produce androstenedione, whereas granulosa-lutein cells greatly increase their capacity to produce progesterone and to convert androstenedione to estradiol. LH and hCG bind to the same LH-hCG receptor. If pregnancy occurs (right panel), human chorionic gonadotropin (hCG) rescues the corpus luteum through their shared LH-hCG receptor. Low-density lipoprotein (LDL) is an important source of cholesterol for steroidogenesis. cAMP = cyclic adenosine monophosphate.
After the appearance of LH receptors, the preovulatory granulosa cells begin to secrete small quantities of progesterone. The preovulatory progesterone secretion, although somewhat limited, is believed to exert positive feedback on the estrogen-primed pituitary to either cause or augment LH release. In addition, during the late follicular phase, LH stimulates thecal cell production of androgens, particularly androstenedione, which are then transferred to the adjacent follicles where they are aromatized to estradiol (see Fig. 5-2). During the early follicular phase, granulosa cells also produce inhibin B, which can feed back on the pituitary to inhibit FSH release (Groome, 1996). As the dominant follicle begins to grow, production of estradiol and the inhibins increases and results in a decline of follicular-phase FSH. This drop in FSH levels is responsible for the failure of other follicles to reach preovulatory status—the Graafian follicle stage—during any one cycle. Thus, 95 percent of plasma estradiol produced at this time is secreted by the dominant follicle—the one destined to ovulate. Concurrently, the contralateral ovary is relatively inactive.
Ovulation
The onset of the gonadotropin surge resulting from increasing estrogen secretion by preovulatory follicles is a relatively precise predictor of ovulation. It occurs 34 to 36 hours before ovum release from the follicle (see Fig. 5-1). LH secretion peaks 10 to 12 hours before ovulation and stimulates resumption of meiosis in the ovum and release of the first polar body. Current studies suggest that in response to LH, increased progesterone and prostaglandin production by the cumulus cells, as well as GDF9 and BMP-15 by the oocyte, activates expression of genes critical to formation of a hyaluronan-rich extracellular matrix by the cumulus complex (Richards, 2007). As seen in Figure 5-3, during synthesis of this matrix, cumulus cells lose contact with one another and move outward from the oocyte along the hyaluronan polymer—this process is called expansion. This results in a 20-fold increase in the complex volume along with an LH-induced remodeling of the ovarian extracellular matrix to allow release of the mature oocyte and its surrounding cumulus cells through the surface epithelium. Activation of proteases likely plays a pivotal role in weakening of the follicular basement membrane and ovulation (Curry, 2006; Ny, 2002).
FIGURE 5-3 An ovulated cumulus-oocyte complex. An oocyte is at the center of the complex. Cumulus cells are widely separated from each other in the cumulus layer by the hyaluronan-rich extracellular matrix. (Photograph contributed by Dr. Kevin J. Doody.)
Luteal or Postovulatory Ovarian Phase
Following ovulation, the corpus luteum develops from the dominant or Graafian follicle remains in a process referred to as luteinization. The basement membrane separating the granulosa-lutein and theca-lutein cells breaks down, and by day 2 postovulation, blood vessels and capillaries invade the granulosa cell layer. The rapid neovascularization of the once-avascular granulosa may be due to angiogenic factors that include vascular endothelial growth factor (VEGF) and others produced in response to LH by theca-lutein and granulosa-lutein cells (Albrecht, 2003; Fraser, 2001). During luteinization, these cells undergo hypertrophy and increase their capacity to synthesize hormones.
LH is the primary luteotropic factor responsible for corpus luteum maintenance (Vande Wiele, 1970). Indeed, LH injections can extend the corpus luteum life span in normal women by 2 weeks (Segaloff, 1951). In normal cycling women, the corpus luteum is maintained by low-frequency, high-amplitude LH pulses secreted by gonadotropes in the anterior pituitary (Filicori, 1986).
The hormone secretion pattern of the corpus luteum differs from that of the follicle (see Fig. 5-1). The increased capacity of granulosa-lutein cells to produce progesterone is the result of increased access to considerably more steroidogenic precursors through blood-borne low-density lipoprotein (LDL)-derived cholesterol as depicted in Figure 5-2 (Carr, 1981a). The important role for LDL in progesterone biosynthesis is supported by the observation that women with extremely low LDL cholesterol levels exhibit minimal progesterone secretion during the luteal phase (Illingworth, 1982). In addition, high-density lipoprotein (HDL) may contribute to progesterone production in granulosa-lutein cells (Ragoobir, 2002).
Estrogen levels follow a more complex pattern of secretion. Specifically, just after ovulation, estrogen levels decrease followed by a secondary rise that reaches a peak production of 0.25 mg/day of 17β-estradiol at the midluteal phase. Toward the end of the luteal phase, there is a secondary decline in estradiol production.
Ovarian progesterone production peaks at 25 to 50 mg/day during the midluteal phase. With pregnancy, the corpus luteum continues progesterone production in response to embryonic human chorionic gonadotropin (hCG), which binds to the same receptor as LH (see Fig. 5-2).
The human corpus luteum is a transient endocrine organ that, in the absence of pregnancy, will rapidly regress 9 to 11 days after ovulation via apoptotic cell death (Vaskivuo, 2002). The mechanisms that control luteolysis remain unclear. However, in part, it results from decreased levels of circulating LH in the late luteal phase and decreased LH sensitivity of luteal cells (Duncan, 1996; Filicori, 1986). The role of other factors is less clear, however, prostaglandin F2α (PGF2α) appears to be luteolytic in nonhuman primates (Auletta, 1987; Wentz, 1973). The endocrine effects, consisting of a dramatic drop in circulating estradiol and progesterone levels, are critical for follicular development and ovulation during the next ovarian cycle. In addition, corpus luteum regression and decline in circulating steroid concentrations signal the endometrium to initiate molecular events that lead to menstruation.
Estrogen and Progesterone Action
The fluctuating levels of ovarian steroids are the direct cause of the endometrial cycle. Recent advances in the molecular biology of estrogen and progesterone receptors have greatly improved understanding of their function. The most biologically potent naturally occurring estrogen—17β-estradiol—is secreted by granulosa cells of the dominant follicle and luteinized granulosa cells of the corpus luteum (see Fig. 5-2). Estrogen is the essential hormonal signal on which most events in the normal menstrual cycle depend. Estradiol action is complex and appears to involve two classic nuclear hormone receptors designated estrogen receptor α (ERα) and β (ERβ) (Katzenellenbogen, 2001). These isoforms are the products of separate genes and can exhibit distinct tissue expression. Both estradiol-receptor complexes act as transcriptional factors that become associated with the estrogen response element of specific genes. They share a robust activation by estradiol. However, differences in their binding affinities to other estrogens and their cell-specific expression patterns suggest that ERα and ERβ receptors may have both distinct and overlapping function (Saunders, 2005). Both receptors are expressed in the uterine endometrium (Bombail, 2008; Lecce, 2001). Estrogens function in many cell types to regulate follicular development, uterine receptivity, or blood flow.
Most progesterone actions on the female reproductive tract are mediated through the nuclear hormone receptors, progesterone receptor type A (PR-A) and B (PR-B). Progesterone enters cells by diffusion and in responsive tissues becomes associated with progesterone receptors (Conneely, 2002). Both progesterone receptor isoforms arise from a single gene, are members of the steroid receptor superfamily of transcription factors, and regulate transcription of target genes. These receptors have unique actions. When PR-A and PR-B receptors are coexpressed, it appears that PR-A can inhibit PR-B gene regulation. The endometrial glands and stroma appear to have different expression patterns for progesterone receptors that vary during the menstrual cycle (Mote, 1999). In addition, progesterone can evoke rapid responses such as changes in intracellular free calcium levels that cannot be explained by genomic mechanisms. G-protein-coupled membrane receptors for progesterone have been identified, but their role in the ovarian-endometrium cycle remains to be elucidated (Peluso, 2007).
The Endometrial Cycle
Proliferative or Preovulatory Endometrial Phase
Fluctuations in estrogen and progesterone levels produce striking effects on the reproductive tract, particularly the endometrium. Epithelial—glandular cells; stromal—mesenchymal cells; and blood vessels of the endometrium replicate cyclically in reproductive-aged women at a rapid rate. The endometrium is regenerated during each ovarian–endometrial cycle. The superficial endometrium, termed the functionalis layer, is shed and regenerated from the deeper basalis layer almost 400 times during the reproductive lifetime of most women (Fig. 5-4). There is no other example in humans of such cyclical shedding and regrowth of an entire tissue.
FIGURE 5-4 The endometrium consists of two layers, the functionalis layer and basalis layer. These layers are supplied by the spiral and basal arteries, respectively. Numerous glands also span these layers. As the menstrual cycle progresses, greater coiling of the spiral arteries and increased gland folding can be seen. Near the end of the menstrual cycle (day 27), the coiled arteries constrict, depriving the functionalis layer of its blood supply and leading to necrosis and sloughing of this layer.
Follicular-phase estradiol production is the most important factor in endometrial recovery following menstruation. Although up to two thirds of the functionalis endometrium is fragmented and shed during menstruation, reepithelialization begins even before menstrual bleeding has ceased. By the fifth day of the endometrial cycle—fifth day of menses, the epithelial surface of the endometrium has been restored, and revascularization is in progress. The preovulatory endometrium is characterized by proliferation of glandular, stromal, and vascular endothelial cells. During the early part of the proliferative phase, the endometrium is usually less than 2 mm thick. The glands are narrow, tubular structures that pursue almost a straight and parallel course from the basalis layer toward the endometrial cavity. Mitotic figures, especially in the glandular epithelium, are identified by the fifth cycle day. Mitotic activity in both epithelium and stroma persists until day 16 to 17, or 2 to 3 days after ovulation. Although blood vessels are numerous and prominent, there is no extravascular blood or leukocyte infiltration in the endometrium at this stage.
Clearly, reepithelialization and angiogenesis are important to endometrial bleeding cessation (Chennazhi, 2009; Rogers, 2009). These are dependent on tissue regrowth, which is estrogen regulated. Epithelial cell growth also is regulated in part by epidermal growth factor (EGF) and transforming growth factor α (TGFα). Stromal cell proliferation appears to increase through paracrine and autocrine actions of estrogen and increased local levels of fibroblast growth factor-9 (Tsai, 2002). Estrogens also increase local production of VEGF, which causes angiogenesis through vessel elongation in the basalis (Gargett, 2001; Sugino, 2002).
By the late proliferative phase, the endometrium thickens from both glandular hyperplasia and increased stromal ground substance, which is edema and proteinaceous material. The loose stroma is especially prominent, and the glands in the functionalis layer are widely separated. This is compared with those of the basalis layer, in which the glands are more crowded and the stroma is denser. At midcycle, as ovulation nears, glandular epithelium becomes taller and pseudostratified. The surface epithelial cells acquire numerous microvilli, which increase epithelial surface area, and cilia, which aid in the movement of endometrial secretions during the secretory phase (Ferenczy, 1976).
Dating the menstrual cycle day by endometrial histological criteria is difficult during the proliferative phase because of considerable phase-length variation among women. Specifically, the follicular phase normally may be as short as 5 to 7 days or as long as 21 to 30 days. In contrast, the luteal or secretory postovulatory phase of the cycle is remarkably constant at 12 to 14 days.
Secretory or Postovulatory Endometrial Phase
During the early secretory phase, endometrial dating is based on glandular epithelium histology. After ovulation, the estrogen-primed endometrium responds to rising progesterone levels in a highly predictable manner. By day 17, glycogen accumulates in the basal portion of glandular epithelium, creating subnuclear vacuoles and pseudostratification. This is the first sign of ovulation that is histologically evident. It is likely the result of direct progesterone action through receptors expressed in glandular cells (Mote, 2000). On day 18, vacuoles move to the apical portion of the secretory nonciliated cells. By day 19, these cells begin to secrete glycoprotein and mucopolysaccharide contents into the lumen (Hafez, 1975). Glandular cell mitosis ceases with secretory activity on day 19 due to rising progesterone levels, which antagonize the mitotic effects of estrogen. Estradiol action is also decreased because of glandular expression of the type 2 isoform of 17β-hydroxysteroid dehydrogenase. This converts estradiol to the less active estrone (Casey, 1996).
Dating in the mid- to late-secretory phase relies on endometrial stromal changes. On days 21 to 24, the stroma becomes edematous. On days 22 to 25, stromal cells surrounding the spiral arterioles begin to enlarge, and stromal mitosis becomes apparent. Days 23 to 28 are characterized by predecidual cells, which surround spiral arterioles.
An important feature of secretory-phase endometrium between days 22 and 25 is striking changes associated with predecidual transformation of the upper two thirds of the functionalis layer. The glands exhibit extensive coiling, and luminal secretions become visible. Changes within the endometrium also can mark the so-called window of implantation seen on days 20 to 24. Epithelial surface cells show decreased microvilli and cilia but appearance of luminal protrusions on the apical cell surface (Nikas, 2003). These pinopodes are important in preparation for blastocyst implantation. They also coincide with changes in the surface glycocalyx that allow acceptance of a blastocyst (Aplin, 2003).
The secretory phase is also highlighted by the continuing growth and development of the spiral arteries. Boyd and Hamilton (1970) emphasized the extraordinary importance of the endometrial spiral or coiled arteries. They arise from the radial arteries, which are myometrial branches of the arcuate and ultimately, uterine vessels (see Fig. 5-4). The morphological and functional properties of spiral arteries are unique and essential for establishing blood flow changes to permit either menstruation or implantation. During endometrial growth, spiral arteries lengthen at a rate appreciably greater than the rate of endometrial tissue thickening (Fig. 5-5). This growth discordance obliges even greater coiling of the already spiraling vessels. Spiral artery development reflects a marked induction of angiogenesis, consisting of widespread vessel sprouting and extension. Perrot-Applanat and associates (1988) described progesterone and estrogen receptors in the smooth muscle cells of the uterus and spiral arteries. Such rapid angiogenesis is regulated, in part, through estrogen- and progesterone-regulated synthesis of VEGF (Ancelin, 2002; Chennazhi, 2009).
FIGURE 5-5 The spiral arteries of human endometrium are modified during the ovulatory cycle. Initially, blood flow changes through these vessels aid endometrial growth. Excessive coiling and blood flow stasis coincide with regression of corpus luteum function and lead to a decline in endometrial tissue volume. Finally, spiral artery coiling leads to endometrial hypoxia and necrosis. Before endometrial bleeding, intense spiral artery vasospasm serves to limit blood loss during menses.
Menstruation
The midluteal–secretory phase of the endometrial cycle is a critical branch point in endometrial development and differentiation. With corpus luteum rescue and continued progesterone secretion, the decidualization process continues. If luteal progesterone production decreases with luteolysis, events leading to menstruation are initiated (Critchley, 2006; Thiruchelvam, 2013).
A notable histological characteristic of late premenstrual-phase endometrium is stromal infiltration by neutrophils, giving a pseudoinflammatory appearance to the tissue. These cells infiltrate primarily on the day or two immediately preceding menses onset. The endometrial stromal and epithelial cells produce interleukin-8 (IL-8), a chemotactic–activating factor for neutrophils (Arici, 1993). Similarly, monocyte chemotactic protein-1 (MCP-1) is synthesized by endometrium and promotes monocyte recruitment (Arici, 1995).
Leukocyte infiltration is considered key to both endometrial extracellular matrix breakdown and repair of the functionalis layer. The term “inflammatory tightrope” refers to the ability of macrophages to assume phenotypes that vary from proinflammatory and phagocytic to immunosuppressive and reparative. These are likely relevant to menstruation, in which tissue breakdown and restoration occur simultaneously (Evans, 2012). Invading leukocytes secrete enzymes that are members of the matrix metalloprotease (MMP) family. These add to the proteases already produced by endometrial stromal cells and effectively initiate matrix degradation. This phenomenon has been proposed to initiate the events leading to menstruation (Dong, 2002). As tissue shedding is completed, microenvironment-regulated changes in macrophage phenotype promote repair and resolution (Evans, 2012; Thiruchelvam, 2013).
Anatomical Events During Menstruation. The classic study by Markee (1940) described tissue and vascular changes in endometrium before menstruation. First, there are marked changes in endometrial blood flow essential for menstruation. With endometrial regression, spiral artery coiling becomes sufficiently severe that resistance to blood flow increases strikingly, causing endometrial hypoxia. Resultant stasis is the primary cause of endometrial ischemia and tissue degeneration (see Fig. 5-5). Vasoconstriction precedes menstruation and is the most striking and constant event observed in the cycle. Intense spiral artery vasoconstriction also serves to limit menstrual blood loss. Blood flow appears to be regulated in an endocrine manner by sex steroid hormone–induced modifications of a paracrine-mediated vasoactive peptide system as described subsequently.
Prostaglandins and Menstruation. Progesterone withdrawal increases expression of cyclooxygenase 2 (COX-2), also called prostaglandin synthase 2, to synthesize prostaglandins. Withdrawal also decreases expression of 15-hydroxyprostaglandin dehydrogenase (PGDH), which degrades prostaglandins (Casey, 1980, 1989). The net result is increased prostaglandin production by endometrial stromal cells and increased prostaglandin-receptor density on blood vessels and surrounding cells.
A role for prostaglandins—especially vasoconstricting PGF2α—in menstruation initiation has been suggested (Abel, 2002). Large amounts of prostaglandins are present in menstrual blood. PGF2α administration to nonpregnant women prompts menstruation and symptoms that mimic dysmenorrhea. Painful menstruation is common and likely caused by myometrial contractions and uterine ischemia. This response is believed to be mediated by PGF2α-induced spiral artery vasoconstriction that causes the uppermost endometrial zones to become hypoxic. The hypoxic environment is a potent inducer of angiogenesis and vascular permeability factors such as VEGF. Prostaglandins serve an important function in the event cascade leading to menstruation that includes vasoconstriction, myometrial contractions, and upregulation of proinflammatory responses.
Activation of Lytic Mechanisms. Following vasoconstriction and endometrial cytokine changes, protease activation within stromal cells and leukocyte invasion is required to degrade the endometrial interstitial matrix. Matrix metalloproteases—MMP-1 and MMP-3—are released from stromal cells and may activate other neutrophilic proteases such as MMP-8 and MMP-9.
Origin of Menstrual Blood. Menstrual bleeding is appreciably arterial rather than venous bleeding. Endometrial bleeding appears to follow rupture of a spiral arteriole and consequent hematoma formation. With a hematoma, the superficial endometrium is distended and ruptures. Subsequently, fissures develop in the adjacent functionalis layer, and blood and tissue fragments are sloughed. Hemorrhage stops with arteriolar constriction. Changes that accompany partial tissue necrosis also serve to seal vessel tips.
The endometrial surface is restored by growth of flanges, or collars, that form the everted free ends of the endometrial glands (Markee, 1940). These flanges increase in diameter very rapidly, and epithelial continuity is reestablished by fusion of the edges of these sheets of migrating cells.
Interval between Menses. The modal interval of menstruation is considered to be 28 days, but variation is considerable among women, as well as in the cycle lengths of a given woman. Marked differences in the intervals between menstrual cycles are not necessarily indicative of infertility. Arey (1939) analyzed 12 studies comprising approximately 20,000 calendar records from 1500 women. He concluded that there is no evidence of perfect menstrual cycle regularity. Among average adult women, a third of cycles departed by more than 2 days from the mean of all cycle lengths. In his analysis of 5322 cycles in 485 normal women, an average interval of 28.4 days was estimated.
THE DECIDUA
This is a specialized, highly modified endometrium of pregnancy. It is essential for hemochorial placentation, that is, one in which maternal blood contacts trophoblast. This relationship requires trophoblast invasion, and considerable research has focused on the interaction between decidual cells and invading trophoblasts. Decidualization, that is, transformation of secretory endometrium to decidua, is dependent on estrogen and progesterone and factors secreted by the implanting blastocyst. The special relationship that exists between the decidua and the invading trophoblast seemingly defies the laws of transplantation immunology. The success of this unique semiallograft not only is of great scientific interest but may involve processes that harbor insights leading to more successful transplantation surgery and perhaps even immunological treatment of neoplasia (Billingham, 1986; Lala, 2002).
Decidual Structure
The decidua is classified into three parts based on anatomical location. Decidua directly beneath blastocyst implantation is modified by trophoblast invasion and becomes the decidua basalis. The decidua capsularis overlies the enlarging blastocyst and initially separates the conceptus from the rest of the uterine cavity (Fig. 5-6). This portion is most prominent during the second month of pregnancy and consists of decidual cells covered by a single layer of flattened epithelial cells. Internally, it contacts the avascular, extraembryonic fetal membrane—the chorion laeve. The remainder of the uterus is lined by decidua parietalis. During early pregnancy, there is a space between the decidua capsularis and parietalis because the gestational sac does not fill the entire uterine cavity. By 14 to 16 weeks’ gestation, the expanding sac has enlarged to completely fill the uterine cavity. The resulting apposition of the decidua capsularis and parietalis creates the decidua vera, and the uterine cavity is functionally obliterated.
FIGURE 5-6 Three portions of the decidua—the basalis, capsularis, and parietalis—are illustrated.
In early pregnancy, the decidua begins to thicken, eventually attaining a depth of 5 to 10 mm. With magnification, furrows and numerous small openings, representing the mouths of uterine glands, can be detected. Later in pregnancy, the decidua becomes thinner, presumably because of pressure exerted by the expanding uterine contents.
The decidua parietalis and basalis are composed of three layers. There is a surface or compact zone—zona compacta; a middle portion or spongy zone—zona spongiosa—with remnants of glands and numerous small blood vessels; and a basal zone—zona basalis. The zona compacta and spongiosa together form the zona functionalis. The basal zone remains after delivery and gives rise to new endometrium.
Decidual Reaction
In human pregnancy, the decidual reaction is completed only with blastocyst implantation. Predecidual changes, however, commence first during the midluteal phase in endometrial stromal cells adjacent to the spiral arteries and arterioles. Thereafter, they spread in waves throughout the uterine endometrium and then from the implantation site. The endometrial stromal cells enlarge to form polygonal or round decidual cells. The nuclei become round and vesicular, and the cytoplasm becomes clear, slightly basophilic, and surrounded by a translucent membrane. Each mature decidual cell becomes surrounded by a pericellular membrane. Thus, the human decidual cells clearly build walls around themselves and possibly around the fetus. The pericellular matrix surrounding the decidual cells may allow attachment of cytotrophoblasts through cellular adhesion molecules. The cell membrane also may provide decidual cell protection against selected cytotrophoblastic proteases.
Decidual Blood Supply
As a consequence of implantation, the blood supply to the decidua capsularis is lost as the embryo-fetus grows. Blood supply to the decidua parietalis through spiral arteries persists. These arteries retain a smooth-muscle wall and endothelium and thereby remain responsive to vasoactive agents.
In contrast, the spiral arterial system supplying the decidua basalis directly beneath the implanting blastocyst, and ultimately the intervillous space, is altered remarkably. These spiral arterioles and arteries are invaded by cytotrophoblasts. During this process, the vessel walls in the basalis are destroyed. Only a shell without smooth muscle or endothelial cells remains. Importantly, as a result, these vascular conduits of maternal blood—which become the uteroplacental vessels—are not responsive to vasoactive agents. Conversely, the fetal chorionic vessels, which transport blood between the placenta and the fetus, contain smooth muscle and thus do respond to vasoactive agents.
Decidual Histology
Early in pregnancy, the zona spongiosa of the decidua consists of large distended glands, often exhibiting marked hyperplasia and separated by minimal stroma. At first, the glands are lined by typical cylindrical uterine epithelium with abundant secretory activity that contributes to blastocyst nourishment. As pregnancy progresses, the epithelium gradually becomes cuboidal or even flattened and later degenerates and sloughs to a greater extent into the gland lumens. With advanced pregnancy, the glandular elements largely disappear. In comparing the decidua parietalis at 16 weeks’ gestation with the early proliferative endometrium of a nonpregnant woman, there is marked hypertrophy but only slight hyperplasia of the endometrial stroma during decidual transformation.
The decidua basalis contributes to formation of the placental basal plate (Fig. 5-7). It differs histologically from the decidua parietalis in two important respects. First, the spongy zone of the decidua basalis consists mainly of arteries and widely dilated veins, and by term, glands have virtually disappeared. Second, the decidua basalis is invaded by many interstitial trophoblast cells and trophoblastic giant cells. Although most abundant in the decidua, the giant cells commonly penetrate the upper myometrium. Their number and invasiveness can be so extensive as to resemble choriocarcinoma.
FIGURE 5-7 Section through a junction of chorion, villi, and decidua basalis in early first-trimester pregnancy. (Photograph contributed by Dr. Kurt Benirschke.)
The Nitabuch layer is a zone of fibrinoid degeneration in which invading trophoblasts meet the decidua basalis. If the decidua is defective, as in placenta accreta, the Nitabuch layer is usually absent (Chap. 41, p. 804). There is also a more superficial, but inconsistent, deposition of fibrin—Rohr stria—at the bottom of the intervillous space and surrounding the anchoring villi. McCombs and Craig (1964) found that decidual necrosis is a normal phenomenon in the first and probably second trimesters. Thus, necrotic decidua obtained through curettage after spontaneous abortion in the first trimester should not necessarily be interpreted as either a cause or an effect of the pregnancy loss.
Both deciduas contain numerous cell types whose composition varies with gestational stage (Loke, 1995). The primary cellular components are the true decidual cells, which differentiated from the endometrial stromal cells, and numerous maternal bone marrow–derived cells.
Early in pregnancy, a striking abundance of large, granular lymphocytes termed decidual natural killer (NK) cells are present in the decidua. In peripheral blood, there are two subsets of NK cells. Approximately 90 percent are highly cytolytic. Ten percent show less cytolytic ability but increased cytokine secretion. In contrast to peripheral blood, 95 percent of NK cells in decidua secrete cytokines, and about half of these unique cells also express angiogenic factors. These decidua NK cells likely play an important role in trophoblast invasion and vasculogenesis.
Decidual Prolactin
In addition to placental development, the decidua potentially provides other functions. The decidua is the source of prolactin that is present in enormous amounts in amnionic fluid (Golander, 1978; Riddick, 1979). Decidual prolactin is not to be confused with placental lactogen (hPL), which is produced only by syncytiotrophoblast. Rather, decidual prolactin is a product of the same gene that encodes for anterior pituitary prolactin. And although the amino-acid sequence of prolactin in both tissues is identical, an alternative promoter is used within the prolactin gene to initiate transcription in decidua (Telgmann, 1998). This may explain the different mechanisms that regulate expression in the decidua versus pituitary (Christian, 2002a,b).
Prolactin preferentially enters amnionic fluid, and little enters maternal blood. Consequently, prolactin levels in amnionic fluid are extraordinarily high and may reach 10,000 ng/mL at 20 to 24 weeks’ gestation (Tyson, 1972). This compares with fetal serum levels of 350 ng/mL and maternal serum levels of 150 to 200 ng/mL. As a result, decidual prolactin is a classic example of paracrine function between maternal and fetal tissues.
The exact physiological roles of decidual prolactin are still unknown. Its action is mediated by the relative expression of two unique prolactin receptors and by the amount of intact or full-length prolactin protein compared with the truncated 16-kDa form (Jabbour, 2001). Receptor expression has been demonstrated in decidua, chorionic cytotrophoblasts, amnionic epithelium, and syncytiotrophoblast (Maaskant, 1996). There are several possible roles for decidual prolactin. First, most or all of this protein hormone enters amnionic fluid. Thus, it may serve in transmembrane solute and water transport and in amnionic fluid volume maintenance. Second, there are prolactin receptors in several bone marrow-derived immune cells, and prolactin may stimulate T cells in an autocrine or paracrine manner (Pellegrini, 1992). This raises the possibility that decidual prolactin may act in regulating immunological functions during pregnancy. Prolactin may play a role in angiogenesis regulation during implantation. Last, decidual prolactin has been shown in the mouse to have a protective function by repressing expression of genes detrimental to pregnancy maintenance (Bao, 2007).
Regulation of decidual prolactin is not clearly defined. Most agents known to inhibit or stimulate pituitary prolactin secretion—including dopamine, dopamine agonists, and thyrotropin-releasing hormone—do not alter decidual prolactin secretion either in vivo or in vitro. Brosens and colleagues (2000) demonstrated that progestins act synergistically with cyclic adenosine monophosphate on endometrial stromal cells in culture to increase prolactin expression. This suggests that the level of progesterone receptor expression may determine the decidualization process, at least as marked by prolactin production. Conversely, various cytokines and growth factors—endothelin-1, IL-1, IL-2, and epidermal growth factor—decrease decidual prolactin secretion (Chao, 1994; Frank, 1995). Studies in decidualized human endometrial cells in culture have led to identification of several transcription factors that regulate decidual prolactin (Jiang, 2011; Lynch, 2009).
IMPLANTATION AND EARLY TROPHOBLAST FORMATION
The fetus is dependent on the placenta for pulmonary, hepatic, and renal functions. These are accomplished through the unique anatomical relationship of the placenta and its uterine interface. Maternal blood spurts from uteroplacental vessels into the placental intervillous space and bathes the outer syncytiotrophoblast. This allows exchange of gases, nutrients, and other substances with fetal capillary blood within the villous core. Thus, fetal and maternal blood are not normally mixed in this hemochorial placenta. There is also a paracrine system that links mother and fetus through the anatomical and biochemical juxtaposition of the maternal decidua parietalis and the extraembryonic chorion laeve, which is fetal. This is an extraordinarily important arrangement for communication between fetus and mother and for maternal immunological acceptance of the conceptus (Guzeloglu-Kayisli, 2009).
Fertilization and Implantation
With ovulation, the secondary oocyte and adhered cells of the cumulus-oocyte complex are freed from the ovary. Although technically this mass of cells is released into the peritoneal cavity, the oocyte is quickly engulfed by the fallopian tube infundibulum. Further transport through the tube is accomplished by directional movement of cilia and tubal peristalsis. Fertilization, which normally occurs in the oviduct, must take place within a few hours, and no more than a day after ovulation. Because of this narrow opportunity window, spermatozoa must be present in the fallopian tube at the time of oocyte arrival. Almost all pregnancies result when intercourse occurs during the 2 days preceding or on the day of ovulation. Thus, postovulatory and postfertilization developmental ages are similar.
Steps of fertilization are highly complex. Molecular mechanisms allow spermatozoa to pass between follicular cells; through the zona pellucida, which is a thick glycoprotein layer surrounding the oocyte cell membrane; and into the oocyte cytoplasm. Fusion of the two nuclei and intermingling of maternal and paternal chromosomes creates the zygote. These steps are reviewed by Primakoff and Myles (2002).
Early human development is described by days or weeks postfertilization, that is, post-conceptional. By contrast, in most chapters of this book, clinical pregnancy dating is calculated from the start of the last menses. As discussed earlier, the follicular phase length is more variable than the luteal phase. Thus, 1 week postfertilization corresponds to approximately 3 weeks from the last menstrual period in women with regular 28-day cycles.
The Zygote
After fertilization, the zygote—a diploid cell with 46 chromosomes—undergoes cleavage, and zygote cells produced by this division are called blastomeres (Fig. 5-8). In the two-cell zygote, the blastomeres and polar body continue to be surrounded by the zona pellucida. The zygote undergoes slow cleavage for 3 days while still within the fallopian tube. As the blastomeres continue to divide, a solid mulberry-like ball of cells—the morula—is produced. The morula enters the uterine cavity about 3 days after fertilization. Gradual accumulation of fluid between the morula cells leads to formation of the early blastocyst.
FIGURE 5-8 Zygote cleavage and blastocyst formation. The morula period begins at the 12- to 16-cell stage and ends when the blastocyst forms, which occurs when there are 50 to 60 blastomeres present. The polar bodies, shown in the 2-cell stage, are small nonfunctional cells that soon degenerate.
The Blastocyst
As early as 4 to 5 days after fertilization, the 58-cell blastula differentiates into five embryo-producing cells—the inner cell mass, and 53 cells destined to form trophoblast (Hertig, 1962). In a 58-cell blastocyst, the outer cells, called the trophectoderm, can be distinguished from the inner cell mass that forms the embryo (see Fig. 5-8).
Interestingly, the 107-cell blastocyst is found to be no larger than the earlier cleavage stages, despite the accumulated fluid. It measures approximately 0.155 mm in diameter, which is similar to the size of the initial postfertilization zygote. At this stage, the eight formative, embryo-producing cells are surrounded by 99 trophoblastic cells. And, the blastocyst is released from the zona pellucida secondary to secretion of specific proteases from the secretory-phase endometrial glands (O’Sullivan, 2002).
Release from the zona pellucida allows blastocyst-produced cytokines and hormones to directly influence endometrial receptivity (Lindhard, 2002). IL-1α and IL-1β are secreted by the blastocyst, and these cytokines likely directly influence the endometrium. Embryos also have been shown to secrete hCG, which may influence endometrial receptivity (Licht, 2001; Lobo, 2001). The receptive endometrium is thought to respond by producing leukemia inhibitory factor (LIF) and colony-stimulating factor-1 (CSF-1). These serve to increase trophoblast protease production. This degrades selected endometrial extracellular matrix proteins and allows trophoblast invasion. Thus, embryo “hatching” is a critical step toward successful pregnancy as it allows association of trophoblasts with endometrial epithelial cells and permits release of trophoblast-produced hormones into the uterine cavity.
Blastocyst Implantation
Six or 7 days after fertilization, the embryo implants the uterine wall. This process can be divided into three phases: (1) apposition—initial contact of the blastocyst to the uterine wall; (2) adhesion—increased physical contact between the blastocyst and uterine epithelium; and (3) invasion—penetration and invasion of syncytiotrophoblast and cytotrophoblasts into the endometrium, inner third of the myometrium, and uterine vasculature.
Successful implantation requires a receptive endometrium appropriately primed with estrogen and progesterone by the corpus luteum. Such uterine receptivity is limited to days 20 to 24 of the cycle. Adherence is mediated by cell-surface receptors at the implantation site that interact with blastocyst receptors (Carson, 2002; Lessey, 2002; Lindhard, 2002; Paria, 2002). If the blastocyst approaches the endometrium after cycle day 24, the potential for adhesion is diminished because antiadhesive glycoprotein synthesis prevents receptor interactions (Navot, 1991).
At the time of its interaction with the endometrium, the blastocyst is composed of 100 to 250 cells. The blastocyst loosely adheres to the endometrial epithelium by apposition. This most commonly occurs on the upper posterior uterine wall. Attachment of the blastocyst trophectoderm to the endometrial surface by apposition and adherence appears to be closely regulated by paracrine interactions between these two tissues.
Successful endometrial blastocyst adhesion involves modification in expression of cellular adhesion molecules (CAMs). The integrins—one of four families of CAMs—are cell-surface receptors that mediate cell adhesion to extracellular matrix proteins (Lessey, 2002). Great diversity of cell binding to several different extracellular matrix proteins is possible by differential regulation of the integrin receptors. Endometrial integrins are hormonally regulated, and a specific set of integrins is expressed at implantation (Lessey, 1996). Specifically, αVβ3 and α4β1 integrins expressed on endometrial epithelium are considered a receptivity marker for blastocyst attachment. Aberrant expression of αVβ3 has been associated with infertility (Lessey, 1995). Recognition-site blockade on integrins for binding to extracellular matrix molecules such as fibronectin will prevent blastocyst attachment (Kaneko, 2013).
The Trophoblast
Human placental formation begins with the trophectoderm, which appears at the morula stage. It gives rise to a trophoblast cell layer encircling the blastocyst. From then until term, the trophoblast plays a critical part at the fetal-maternal interface. Trophoblast exhibits the most variable structure, function, and developmental pattern of all placental components. Its invasiveness promotes implantation, its nutritional role for the conceptus is reflected in its name, and its endocrine organ function is essential to maternal physiological adaptations and to pregnancy maintenance.
Trophoblast Differentiation
By the eighth day postfertilization, after initial implantation, the trophoblast has differentiated into an outer multinucleated syncytium—primitive syncytiotrophoblast, and an inner layer of primitive mononuclear cells—cytotrophoblasts (Fig. 5-9). The latter are germinal cells for the syncytium. Each cytotrophoblast has a well-demarcated cell border, a single nucleus, and ability to undergo DNA synthesis and mitosis (Arnholdt, 1991). These are lacking in the syncytiotrophoblast. It is so named because instead of individual cells, it has an amorphous cytoplasm without cell borders, nuclei that are multiple and diverse in size and shape, and a continuous syncytial lining. This configuration aids transport.
FIGURE 5-9 Drawing of sections through implanted blastocysts. A. At 10 days. B. At 12 days after fertilization. This stage is characterized by the intercommunication of the lacunae filled with maternal blood. Note in (B) that large cavities have appeared in the extraembryonic mesoderm, forming the beginning of the extraembryonic coelom. Also note that extraembryonic endodermal cells have begun to form on the inside of the primitive yolk sac. (Adapted from Moore, 1988.)
After implantation is complete, trophoblast further differentiate along two main pathways, giving rise to villous and extravillous trophoblast. As shown in Figure 5-10, both pathways create populations of trophoblast cells that have distinct functions (Loke, 1995). The villous trophoblast gives rise to the chorionic villi, which primarily transport oxygen, nutrients, and other compounds between the fetus and mother. Extravillous trophoblasts migrate into the decidua and myometrium and also penetrate maternal vasculature, thus coming into contact with various maternal cell types (Pijnenborg, 1994). Extravillous trophoblasts are thus further classified as interstitial trophoblasts and endovascular trophoblasts. The interstitial trophoblasts invade the decidua and eventually penetrate the myometrium to form placental bed giant cells. These trophoblasts also surround spiral arteries. The endovascular trophoblasts penetrate the spiral artery lumens (Pijnenborg, 1983). These are both discussed in greater detail in subsequent sections.
FIGURE 5-10 Extravillous trophoblasts are found outside the villus and can be subdivided into endovascular and interstitial categories. Endovascular trophoblasts invade and transform spiral arteries during pregnancy to create low-resistance blood flow that is characteristic of the placenta. Interstitial trophoblasts invade the decidua and surround spiral arteries.
Early Trophoblast Invasion
After gentle erosion between epithelial cells of the surface endometrium, invading trophoblasts burrow deeper. By the 10th day, the blastocyst becomes totally encased within the endometrium. The mechanisms leading to trophoblast invasion are similar to those of metastasizing malignant cells and are discussed further on page 93.
At 9 days of development, the blastocyst wall facing the uterine lumen is a single layer of flattened cells (see Fig. 5-9A). The opposite, thicker wall comprises two zones—the trophoblasts and the embryo-forming inner cell mass. As early as 7½ days after fertilization, the inner cell mass or embryonic disc is differentiated into a thick plate of primitive ectoderm and an underlying layer of endoderm. Some small cells appear between the embryonic disc and the trophoblast and enclose a space that will become the amnionic cavity.
Embryonic mesenchyme first appears as isolated cells within the blastocyst cavity. When the cavity is completely lined with this mesoderm, it is termed the chorionic vesicle, and its membrane, now called the chorion, is composed of trophoblasts and mesenchyme. Some mesenchymal cells eventually will condense to form the body stalk. This stalk joins the embryo to the nutrient chorion and later develops into the umbilical cord. The body stalk can be recognized at an early stage at the caudal end of the embryonic disc (Fig. 7-4, 129).
Lacunae Formation within the Syncytiotrophoblast
As the embryo enlarges, more maternal decidua basalis is invaded by syncytiotrophoblast. Beginning approximately 12 days after conception, the syncytiotrophoblast is permeated by a system of intercommunicating channels called trophoblastic lacunae. After invasion of superficial decidual capillary walls, lacunae become filled with maternal blood (see Figs. 5-9B and 5-11). At the same time, the decidual reaction intensifies in the surrounding stroma. This is characterized by decidual stromal cell enlargement and glycogen storage.
FIGURE 5-11 Early implantation of a conceptus. (Photograph contributed by Dr. Kurt Benirschke.)
Placental Organization
Chorionic Villi
With deeper blastocyst invasion into the decidua, the extravillous cytotrophoblasts give rise to solid primary villi composed of a cytotrophoblast core covered by syncytiotrophoblast. These arise from buds of cytotrophoblast that protrude into the primitive syncytium before 12 days postfertilization. As the lacunae join, a complicated labyrinth is formed that is partitioned by these solid cytotrophoblastic columns. The trophoblast-lined labyrinthine channels form the intervillous space, and the solid cellular columns form the primary villous stalks. The villi initially are located over the entire blastocyst surface. They later disappear except over the most deeply implanted portion, which is destined to form the placenta.
Beginning on approximately the 12th day after fertilization, mesenchymal cords derived from extraembryonic mesoderm invade the solid trophoblast columns. These form secondary villi. Once angiogenesis begins in the mesenchymal cores, tertiary villi are formed. Although maternal venous sinuses are tapped early in implantation, maternal arterial blood does not enter the intervillous space until around day 15. By approximately the 17th day, however, fetal blood vessels are functional, and a placental circulation is established. The fetal–placental circulation is completed when the blood vessels of the embryo are connected with chorionic vessels. In some villi, angiogenesis fails from lack of circulation. They can be seen normally, but the most striking exaggeration of this process is seen with hydatidiform mole (Fig. 20-1, p. 397).
Villi are covered by an outer layer of syncytium and an inner layer of cytotrophoblasts, which are also known as Langhans cells. Cytotrophoblast proliferation at the villous tips produces the trophoblastic cell columns that form anchoring villi. They are not invaded by fetal mesenchyme, and they are anchored to the decidua at the basal plate. Thus, the base of the intervillous space faces the maternal side and consists of cytotrophoblasts from cell columns, the covering shell of syncytiotrophoblast, and maternal decidua of the basal plate. The base of the chorionic plate forms the roof of the intervillous space. It consists of two layers of trophoblasts externally and fibrous mesoderm internally. The “definitive” chorionic plate is formed by 8 to 10 weeks as the amnionic and primary chorionic plate mesenchyme fuse together. This formation is accomplished by expansion of the amnionic sac, which also surrounds the connective stalk and the allantois and joins these structures to form the umbilical cord (Kaufmann, 1992).
Villus Ultrastructure
Interpretation of the fine structure of the placenta came from electron microscopic studies of Wislocki and Dempsey (1955). There are prominent microvilli on the syncytial surface that correspond to the so-called brush border described by light microscopy. Associated pinocytotic vacuoles and vesicles are related to absorptive and secretory placental functions. Microvilli act to increase surface area in direct contact with maternal blood. This contact between the trophoblast and maternal blood is the defining characteristic of a hemochorial placenta.
The human hemochorial placenta can be subdivided into hemodichorial or hemomonochorial (Enders, 1965). The dichorial type is more prominent during the first trimester of gestation. It consists of the inner layer of the cytotrophoblasts and associated basal lamina, covered by a syncytiotrophoblast layer. Later in gestation the inner layer of cytotrophoblasts is no longer continuous, and by term, there are only scattered cells present (Fig. 5-12). These create a narrower hemomonochorial barrier that aids nutrient and oxygen transport to the fetus.
FIGURE 5-12 Electron micrograph of term human placenta villus. A villus capillary filled with red blood cells (asterisks) is seen in close proximity to the microvilli border. (From Boyd, 1970, with permission.)
PLACENTA AND CHORION DEVELOPMENT
Chorion and Decidua Development
In early pregnancy, the villi are distributed over the entire periphery of the chorionic membrane (Fig. 5-13). As the blastocyst with its surrounding trophoblasts grows and expands into the decidua, one pole faces the endometrial cavity. The opposite pole will form the placenta from villous trophoblasts and anchoring cytotrophoblasts. Chorionic villi in contact with the decidua basalis proliferate to form the chorion frondosum—or leafy chorion—which is the fetal component of the placenta. As growth of embryonic and extraembryonic tissues continues, the blood supply to the chorion facing the endometrial cavity is restricted. Because of this, villi in contact with the decidua capsularis cease to grow and then degenerate. This portion of the chorion becomes the avascular fetal membrane that abuts the decidua parietalis, that is, the chorion laeve—or smooth chorion. This smooth chorion is composed of cytotrophoblasts and fetal mesodermal mesenchyme that survives in a relatively low-oxygen atmosphere.
FIGURE 5-13 Photograph of an opened chorionic sac. An early embryo and yolk sac are seen. Note the prominent fringe of chorionic villi. (From Boyd, 1970, with permission.)
Until near the end of the third month, the chorion laeve is separated from the amnion by the exocoelomic cavity. Thereafter, they are in intimate contact to form an avascular amniochorion. The chorion laeve is generally more translucent than the amnion and rarely exceeds 1-mm thickness. These two structures are important sites of molecular transfer and metabolic activity. Moreover, they constitute an important paracrine arm of the fetal–maternal communication system.
Maternal Regulation of Trophoblast Invasion and Vascular Growth
During the first half of pregnancy, decidual natural killer cells (dNK) accumulate in the decidua and are found in direct contact with trophoblasts. As described on page 88, these cells lack cytotoxic functions and are able to dampen inflammatory T(H)17 cells. These along with other unique properties distinguish dNK cells from circulating natural killer cells and from natural killer cells in the endometrium before pregnancy (Fu, 2013; Winger, 2013). Recent studies suggest that decidual macrophages play a regulatory role in inhibiting NK cell killing during pregnancy (Co, 2013). This importantly prevents them from recognizing and destroying fetal cells as “foreign.” Hanna and colleagues (2006) have elucidated the ability of dNK cells to attract and promote trophoblast invasion into the decidua and promote vascular growth. Decidual NK cells express both IL-8 and interferon-inducible protein-10, which bind to receptors on invasive trophoblast cells to promote their decidual invasion toward the spiral arteries. Decidual NK cells also produce proangiogenic factors, including VEGF and placental growth factor (PlGF), which promote vascular growth in the decidua. In addition, trophoblasts secrete specific chemokines that attract the dNK cells to the maternal-fetal interface. Thus, both cell types simultaneously attract each other.
Trophoblast Invasion of the Endometrium
Extravillous trophoblasts of the first-trimester placenta are highly invasive. They form cell columns that extend from the endometrium to the inner third of the myometrium. Recall that hemochorial placental development requires invasion of endometrium and spiral arteries. This process occurs under low-oxygen conditions, and regulatory factors that are induced under hypoxic conditions contribute in part to invasive trophoblast activation (Soares, 2012). Invasive trophoblasts secrete numerous proteolytic enzymes that digest extracellular matrix and activate proteinases already present in the endometrium. Trophoblasts produce urokinase-type plasminogen activator, which converts plasminogen into the broadly acting serine protease, plasmin. This in turn both degrades matrix proteins and activates matrix metalloproteases. One member of the MMP family, MMP-9, appears to be critical for human trophoblast invasion. The timing and extent of trophoblast invasion is regulated by a balanced interplay between pro- and antiinvasive factors.
The relative ability to invade maternal tissue in early pregnancy compared with limited invasiveness in late pregnancy is controlled by autocrine and paracrine trophoblastic and endometrial factors. Trophoblasts secrete insulin-like growth factor II, which acts in an autocrine manner. It promotes invasion into the endometrium, whereas decidual cells secrete insulin-like growth factor binding-protein type 4, which blocks this autocrine loop. Thus, the degree of trophoblast invasion is controlled by matrix degradation regulation and by factors that cause trophoblast migration.
Low estradiol levels in the first trimester are critical for trophoblast invasion and remodeling of the spiral arteries. Recent studies in nonhuman primates suggest that the increase in second-trimester estradiol levels suppresses and limits vessel remodeling by reducing trophoblast expression of VEGF and specific integrin receptors (Bonagura, 2012). As the extravillous trophoblast differentiates, it gains expression of integrin receptors that recognize the extracellular matrix proteins collagen IV, laminin, and fibronectin. Binding of these extracellular matrix proteins to specific integrin receptors initiates signals that promote trophoblast cell migration and differentiation. As pregnancy advances, increasing estradiol levels repress and thus control the extent of uterine vessel transformation via downregulation of VEGF and integrin receptor expression.
Invasion of Spiral Arteries
One of the most remarkable features of human placental development is the extensive modification of maternal vasculature by trophoblasts, which are by definition of fetal origin. These events occur in the first half of pregnancy and are considered in detail because of their importance to uteroplacental blood flow. They are also integral to some pathological conditions such as preeclampsia, fetal-growth restriction, and preterm birth. Spiral artery modifications are carried out by two populations of extravillous trophoblast—interstitial trophoblasts, which surround the arteries, and endovascular trophoblasts, which penetrate the spiral artery lumen (see Fig. 5-10). Although earlier work has focused on the role of the endovascular trophoblast, interstitial trophoblast function has more recently been investigated (Benirschke, 2012; Pijnenborg, 1983). These interstitial cells constitute a major portion of the placental bed and penetrate the decidua and adjacent myometrium. They aggregate around spiral arteries, and their functions may include vessel preparation for endovascular trophoblast invasion.
Hamilton and Boyd (1966) report that Friedlander in 1870 first described structural changes in spiral arteries. Endovascular trophoblasts first enter the spiral-artery lumens and initially form cellular plugs. They then destroy vascular endothelium via an apoptosis mechanism and invade and modify the vascular media. Thus, fibrinoid material replaces smooth muscle and connective tissue of the vessel media. Spiral arteries later regenerate endothelium. Invading endovascular trophoblasts can extend several centimeters along the vessel lumen, and they must migrate against arterial flow. These vascular changes are not observed in the decidua parietalis, that is, in decidual sites removed from the invading cytotrophoblasts. Of note, invasion by trophoblasts involves only the decidual spiral arteries and not decidual veins.
In their summary of uteroplacental vasculature, Ramsey and Donner (1980) described uteroplacental vessel development as proceeding in two waves or stages. The first wave occurs before 12 weeks’ postfertilization and consists of invasion and modification of spiral arteries up to the border between the decidua and myometrium. The second wave is between 12 and 16 weeks and involves some invasion of the intramyometrial segments of spiral arteries. Remodeling converts narrow-lumen, muscular spiral arteries into dilated, low-resistance uteroplacental vessels. Molecular mechanisms of these crucial events, and their significance in the pathogenesis of preeclampsia and fetal-growth restriction, have been reviewed by Kaufmann (2003) and Red-Horse (2006).
Establishment of Maternal Blood Flow
Approximately 1 month after conception, maternal blood enters the intervillous space in fountain-like bursts from the spiral arteries. Blood is propelled outside of the maternal vessels and sweeps over and directly bathes the syncytiotrophoblast. The apical surface of the syncytiotrophoblast consists of a complex microvillous structure that undergoes continual shedding and reformation during pregnancy.
Villus Branching
Although certain villi of the chorion frondosum extend from the chorionic plate to the decidua to serve as anchoring villi, most villi arborize and end freely within the intervillous space. As gestation proceeds, the short, thick, early stem villi branch to form progressively finer subdivisions and greater numbers of increasingly smaller villi (Fig. 5-14). Each of the truncal or main stem villi and their ramifications (rami) constitutes a placental lobule, or cotyledon. Each lobule is supplied with a single truncal branch of the chorionic artery. And each lobule has a single vein so that lobules constitute functional units of placental architecture.
FIGURE 5-14 Electron micrographs (A, C) and photomicrographs (B, D) of early and late human placentas. A and B. Limited branching of villi is seen in this early placenta. C and D. With placenta maturation, increasing villous arborization is seen, and villous capillaries lie closer to the surface of each villus. (Photomicrographs contributed by Dr. Kurt Benirschke. Electron micrographs from King, 1975, with permission.)
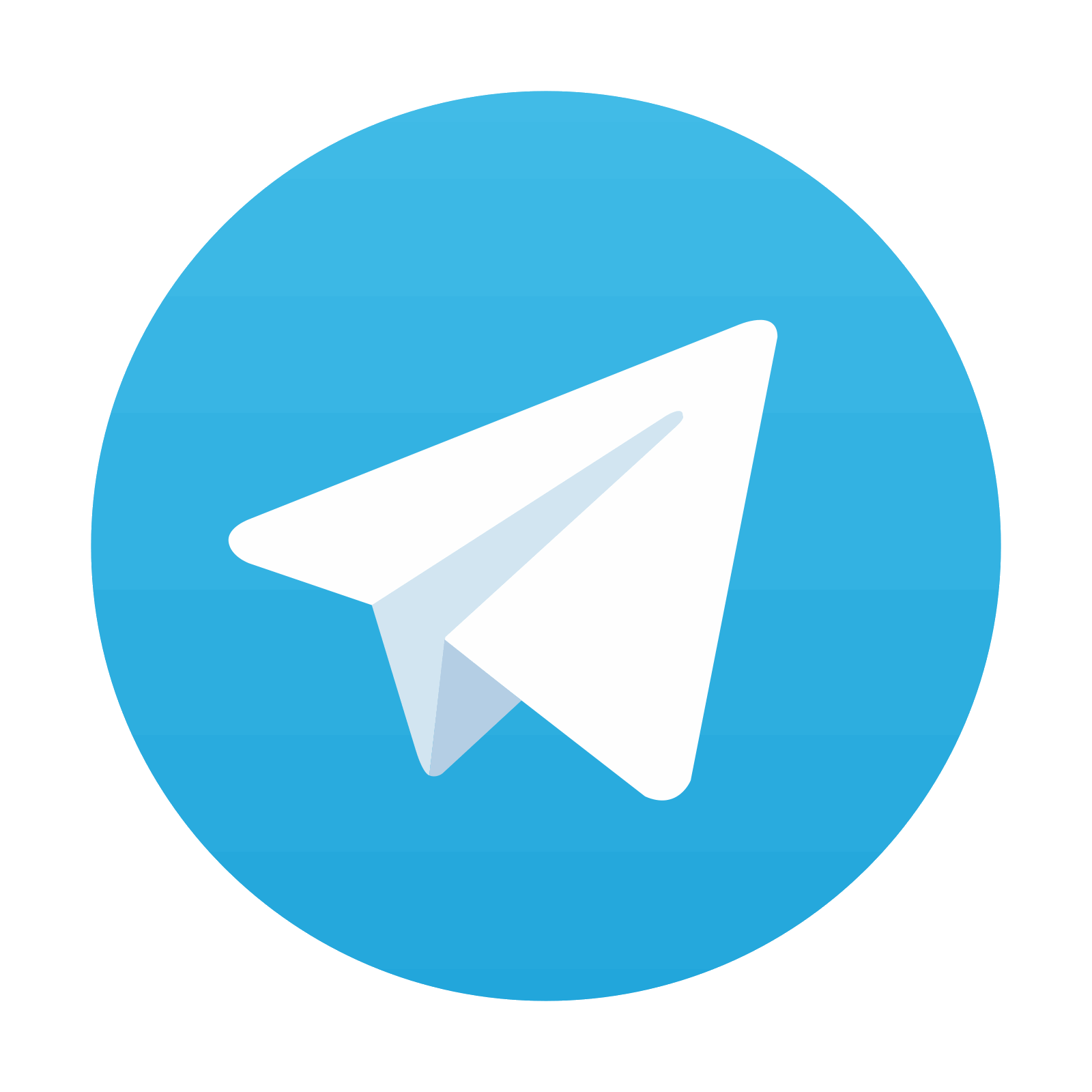
Stay updated, free articles. Join our Telegram channel
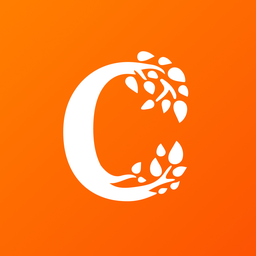
Full access? Get Clinical Tree
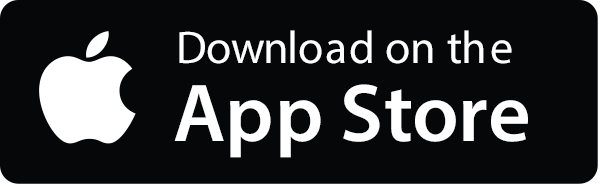
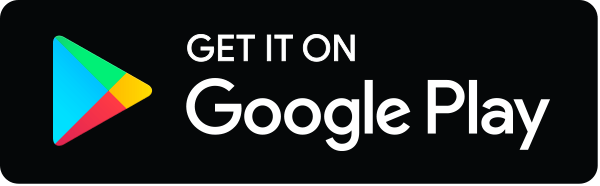