FIGURE 44-1 Increments in fetal weight gain in grams per day from 24 to 42 weeks’ gestation. The black line represents the mean and the outer blue lines depict ±2 standard deviations. Data from pregnancies managed at Parkland Hospital. (Image courtesy of Dr. Don McIntire.)
Fetal development is determined by maternal provision of substrate, placental transfer of these substrates, and fetal growth potential governed by the genome. However, the precise cellular and molecular mechanisms by which normal fetal growth ensues are incompletely understood. That said, there is considerable evidence that insulin and insulin-like growth factors, particularly insulin-like growth factor-I (IGF-I), have an important role in regulation of fetal growth and weight gain (Luo, 2012; Murray, 2013). These growth factors are produced by virtually all fetal organs and are potent stimulators of cell division and differentiation.
Other hormones implicated in fetal growth have been identified in recent years, particularly hormones derived from adipose tissue. These hormones are known broadly as adipokines and include leptin, the protein product of the obesity gene. Fetal leptin concentrations increase during gestation, and they correlate with birthweight (Forhead, 2009; Karakosta, 2011). This relationship, however, is controversial in growth-restricted fetuses (Kyriakakou, 2008; Mise, 2007). Other adipokines under investigation include adiponectin, ghrelin, follistatin, resistin, visfatin, vaspin, omentin-1, apelin, and chemerin. Data for these adipokines are often conflicting, and their roles in normal and disordered fetal growth are still being elucidated (Chap. 48, p. 961).
Fetal growth is also dependent on an adequate supply of nutrients. As discussed in Chapter 4 (p. 53), glucose transfer has been extensively studied during pregnancy. Both excessive and diminished maternal glucose availability affect fetal growth. Reducing maternal glucose levels may result in a lower birthweight. Still, growth-restricted neonates do not typically show pathologically low glucose concentrations in their cord blood (Pardi, 2006). Fetal-growth restriction in response to glucose deprivation generally results only after long-term severe maternal caloric deprivation (Lechtig, 1975).
Excessive glycemia produces macrosomia. Varying levels of glucose affect fetal growth via insulin and its associated insulin-like growth factors discussed earlier. The Hyperglycemia and Adverse Pregnancy Outcomes (HAPO) Study Cooperative Research Group (2008) found that elevated cord C-peptide levels, which reflect fetal hyperinsulinemia, have been associated with increased birthweight. This relationship was noted even in women with maternal glucose levels below the threshold for diabetes. Overgrowth does occur in the fetuses of euglycemic women. Its etiology is thus likely more complicated than a paradigm of dysregulated glucose metabolism resulting in fetal hyperinsulinemia (Catalano, 2011).
Excessive transfer of lipids to the fetus has also been postulated to result in fetal overgrowth (Higa, 2013). Free or nonesterified fatty acids in maternal plasma may be transferred to the fetus via facilitated diffusion or after liberation of fatty acids from triglycerides by trophoblastic lipases (Gil-Sánchez, 2012). Generally speaking, lipolytic activity is increased in pregnancy, and fatty acids have been reported to be increased in nonobese women during the third trimester (Diderholm, 2005). In obese women without diabetes who were fed a controlled diet, neonatal adiposity was strongly linked both with fasting triglyceride levels in early pregnancy and with free fatty acid levels at 26 to 28 weeks’ gestation (Harmon, 2011). Other studies have correlated maternal triglyceride levels with birthweight in both early and late pregnancy (Di Cianni, 2005; Vrijkotte, 2011). Overgrown infants have higher placental levels of certain fatty acids, particularly omega-3, and this has been associated with increased trophoblastic lipase expression (Varastehpour, 2006). Conversely, growth restriction in the third trimester has been associated with decreased maternal lipolysis (Diderholm, 2006). This may be related to dysregulation of the triglyceride lipase gene family, which has been reported in placentas from pregnancies complicated by fetal-growth restriction (Gauster, 2007).
Amino acids undergo active transport from maternal blood to the fetus, which explains the normally higher fetal concentrations. This concentration differential is decreased in growth restriction because of lower fetal amino acid levels and higher maternal amino acid concentrations (Cetin, 1996). The etiology of this altered ratio is uncertain, but there are multiple points at which dysregulation can occur. Amino acids that reach the fetus must first cross the microvillus membrane at the maternal interface, traverse the trophoblastic cell, and finally cross the basal membrane into fetal blood (Chap. 5, p. 92). This process is incompletely understood, particularly with respect to trophoblast metabolism of amino acids and export mechanisms from the trophoblast to the fetus. Jansson and colleagues (2013) have linked expression and activity of particular amino-acid transporters at the microvillous membrane with increasing birthweight and maternal body mass index (BMI). Expression of particular amino acid efflux transporters has been positively correlated with multiple measures of fetal and neonatal growth (Cleal, 2011).
Normal Birthweight
Normative data for fetal growth based on birthweight vary with ethnicity and geographic region. For example, infants born to women who reside at high altitudes are smaller than those born at sea level. Term infants average 3400 g at sea level, 3200 g at 5000 feet, and 2900 g at 10,000 feet. Accordingly, researchers have developed fetal-growth curves using various populations and geographic locations throughout the United States (Brenner, 1976; Ott, 1993; Overpeck, 1999; Williams, 1975). Because these curves are based on specific ethnic or regional groups, they are not representative of the entire population.
To address this, data such as those shown in Table 44-1 were derived on a nationwide basis in both the United States and Canada (Alexander, 1996; Kramer, 2001). Data from more than 3.1 million mothers with singleton liveborn infants in the United States during 1991 were used to derive the growth curve by Alexander and colleagues, colored red in Figure 44-2. Also shown, the previously published regional fetal-growth curve data in general underestimated birthweights compared with national data. Importantly, there are significant ethnic and racial variations in neonatal mortality rates within the national neonatal mortality rate and within national birthweight and gestational age categories (Alexander, 1999, 2003).
TABLE 44-1. Smoothed Percentiles of Birthweight (g) for Gestational Age in the United States Based on 3,134,879 Singleton Live Births
FIGURE 44-2 Comparison of fetal-growth curves for infants born in different regions of the United States and compared with those of the nation at large. (Modified from Alexander, 1996.)
The work of Alexander and associates (1996) is most accurately termed a population reference, rather than a standard. Iams (2010) emphasizes the problems that result from blending a population reference for fetal growth with a fetal-growth standard. A population reference incorporates pregnancies of varying risks, along with the resulting outcomes, both normal and abnormal. In contrast, a standard incorporates normal pregnancies with normal outcomes. Because population references include preterm births, which are more likely to be growth restricted, it has been argued that the associated birthweight data underestimate deficient fetal growth (Mayer, 2013; Zhang, 2010). That said, there is not a widely accepted standard for the United States.
Fetal Growth versus Birthweight
Most of what is known regarding normal and abnormal human fetal growth is actually based on birthweights that are assembled as references for fetal growth at particular gestational ages. This is problematic, however, because birthweight does not define the rate of fetal growth. Indeed, such birthweight curves reveal compromised growth only at the extreme of impaired growth. Thus, they cannot be used to identify the fetus who fails to achieve an expected size but whose birthweight is above the 10th percentile. For example, a fetus with a birthweight in the 40th percentile may not have achieved its genomic growth potential for a birthweight in the 80th percentile. The rate or velocity of fetal growth can be estimated by serial sonographic anthropometry. For example, Milovanovic (2012) demonstrated that the growth rate of intrinsically small-for-gestational age newborns approximates that of appropriate-for-gestational age neonates. Diminished growth velocity has been linked to perinatal morbidity and adverse postnatal metabolic changes that are independent of birthweight (Beltrand, 2008; Owen, 1997, 1998). Conversely, an excessive fetal-growth velocity, particularly of the abdominal circumference—which may be correlated with increased hepatic blood flow—is associated with an overgrown neonate. This is especially true when detected earlier in pregnancy (Ebbing, 2011; Kessler, 2011; Mulder, 2010).
FETAL–GROWTH RESTRICTION
Definition
Low-birthweight newborns who are small for gestational age are often designated as having fetal-growth restriction. In 1963, Lubchenco and coworkers published detailed comparisons of gestational ages with birthweights to derive norms for expected fetal size at a given gestational week. Battaglia and Lubchenco (1967) then classified small-for-gestational-age (SGA) neonates as those whose weights were below the 10th percentile for their gestational age. Such infants were shown to be at increased risk for neonatal death. For example, the neonatal mortality rate of SGA infants born at 38 weeks was 1 percent compared with 0.2 percent in those with appropriate birthweights.
Many infants with birthweights < 10th percentile, however, are not pathologically growth restricted, but are small simply because of normal biological factors. As many as 25 to 60 percent of SGA infants are thought to be appropriately grown when maternal ethnic group, parity, weight, and height are considered (Gardosi, 1992; Manning, 1991). These small but normal infants also do not show evidence of the postnatal metabolic derangements commonly associated with deficient fetal growth. Moreover, intrinsically SGA infants remain significantly smaller during surveillance to 2 years compared with appropriate-for-gestational age neonates, but they do not show differences in measures of metabolic risk (Milovanovic, 2012).
Because of these disparities, other classifications have been developed. Seeds (1984) suggested a definition based on birthweight < 5th percentile. Usher and McLean (1969) suggested that fetal-growth standards should be based on mean weights-for-age, with normal limits defined by ±2 standard deviations. This definition would limit SGA infants to 3 percent of births instead of 10 percent. In a population-based analysis of 122,754 births at Parkland Hospital, McIntire and colleagues (1999) showed this definition to be clinically meaningful. Also, as shown in Figure 44-3, most adverse outcomes are in infants < 3rd percentile.
FIGURE 44-3 Relationship between birthweight percentile and perinatal mortality and morbidity rates in 1560 small-for-gestational age fetuses. A progressive increase in both mortality and morbidity rates is observed as birthweight percentile falls. (Data from Manning, 1995.)
More recently, individual or customized fetal-growth potential has been proposed in place of a population-based cutoff. In this model, a fetus that deviates from its individual optimal size at a given gestational age is considered either overgrown or growth restricted (Bukowski, 2008). Such optimal projections are based on maternal race or ethnicity. However, the superiority of customized growth curves has not been established (Hutcheon, 2011a,b; Larkin, 2012; Zhang, 2011).
Symmetrical versus Asymmetrical Growth Restriction
Campbell and Thoms (1977) described the use of the sonographically determined head-to-abdomen circumference ratio (HC/AC) to differentiate growth-restricted fetuses. Those who were symmetrical were proportionately small, and those who were asymmetrical had disproportionately lagging abdominal growth. Furthermore, the onset or etiology of a particular fetal insult has been hypothetically linked to either type of growth restriction. In the instance of symmetrical growth restriction, an early insult could result in a relative decrease in cell number and size. For example, global insults such as from chemical exposure, viral infection, or cellular maldevelopment with aneuploidy may cause a proportionate reduction of both head and body size. Asymmetrical growth restriction might follow a late pregnancy insult such as placental insufficiency from hypertension. Resultant diminished glucose transfer and hepatic storage would primarily affect cell size and not number, and fetal abdominal circumference—which reflects liver size—would be reduced. Such somatic-growth restriction is proposed to result from preferential shunting of oxygen and nutrients to the brain. This allows normal brain and head growth, that is—brain sparing. Accordingly, the ratio of brain weight to liver weight during the last 12 weeks—usually about 3 to 1—may be increased to 5 to 1 or more in severely growth-restricted infants.
Because of brain-sparing effects, asymmetrical fetuses were thought to be preferentially protected from the full effects of growth restriction. Considerable evidence has since accrued that fetal-growth patterns are much more complex. For example, Nicolaides and coworkers (1991) observed that fetuses with aneuploidy typically had disproportionately large head sizes and thus were asymmetrically growth restricted, which was contrary to contemporaneous thinking. Moreover, most preterm infants with growth restriction due to preeclampsia and associated uteroplacental insufficiency were found to have more symmetrical growth impairment—again, a departure from accepted principles (Salafia, 1995).
More evidence of the complexity of growth patterns was presented by Dashe and associates (2000). These investigators analyzed 8722 consecutive liveborn singletons who had undergone sonographic examination within 4 weeks of delivery. Although only 20 percent of growth-restricted fetuses demonstrated sonographic head-to-abdomen asymmetry, these fetuses were at increased risk for intrapartum and neonatal complications. Symmetrically growth-restricted fetuses were not at increased risk for adverse outcomes compared with those appropriately grown. These investigators concluded that asymmetrical fetal-growth restriction represented significantly disordered growth, whereas symmetrical growth restriction more likely represented normal, genetically determined small stature.
Finally, data from Holland further challenge the concept of “brain sparing.” Roza and associates (2008) provided surveillance of 935 Rotterdam toddlers enrolled between 2003 and 2007 in the Generation R Study. Using the Child Behavior Checklist at age 18 months, they found that infants with circulatory redistribution—brain sparing—had a higher incidence of behavioral problems. In another study, evidence of brain sparing was found in half of 62 growth-restricted fetuses with birthweight < 10th percentile and who showed abnormal umbilical artery Doppler flow studies (Figueras, 2011a). Compared with controls, these neonates had significantly lower neurobehavioral scores in multiple areas, suggesting profound brain injury.
Placental Abnormalities
Fetal-growth restriction was included by Brosens and colleagues (2011) as one of the “great obstetrical syndromes” associated with defects in early placentation. Rogers and coworkers (1999) had observed that implantation site disorders such as incomplete trophoblastic invasion are associated with both fetal-growth restriction and hypertensive disorders. They concluded that implantation site disorders may be both a cause and consequence of hypoperfusion at the placental site. These disorders ultimately lead to pregnancy complications such as fetal-growth restriction with or without maternal hypertension. This comports with the association of certain placental angiogenic factors with pregnancy hypertensive disorders (Chap. 40, p. 735). Thus, it may be that placentas from pregnancies complicated by hypertension elaborate these angiogenic factors in response to placental site hypoperfusion, whereas pregnancies complicated by fetal-growth restriction without hypertension do not (Jeyabalan, 2008).
Mechanisms leading to abnormal trophoblastic invasion are likely multifactorial, and both vascular and immunological etiologies have been proposed. Recently, atrial natriuretic peptide converting enzyme, also known as corrin, has been shown to play a critical role in trophoblastic invasion and remodeling of the uterine spiral arteries. These processes are impaired in corrin-deficient mice, which also develop evidence of preeclampsia. Moreover, mutations in the gene for corrin have also been reported in women with preeclampsia (Cui, 2012).
Notably, several immunological abnormalities have been associated with fetal-growth restriction. This raises the prospect of maternal rejection of the “paternal semiallograft.” Rudzinski and colleagues (2013) studied C4d, a component of complement that is associated with humoral rejection of transplanted tissues. They found this to be highly associated with chronic villitis—88 percent of cases versus only 5 percent of controls—and with reduced placental weight. Greer and associates (2012) studied 10,204 placentas and reported that chronic villitis was associated with placental hypoperfusion, fetal acidemia, and fetal-growth restriction and its sequelae. Kovo and coworkers (2010) found that chronic villitis is more strongly associated with fetal-growth restriction than with preeclampsia. Redline (2007) described the findings of activated maternal lymphocytes among fetal trophoblast. However, this author notes uncertainty as to whether these pathological changes represent maternal immunological rejection.
Morbidity and Mortality
Perinatal Risk
As shown in Figure 44-3, fetal-growth restriction is associated with substantive perinatal morbidity and mortality rates. Rates of stillbirth, birth asphyxia, meconium aspiration, and neonatal hypoglycemia and hypothermia are all increased, as is the prevalence of abnormal neurological development (Jacobsson, 2008; Paz, 1995). This is true for both term and preterm growth-restricted infants (McIntire, 1999; Wu, 2006). Smulian and colleagues (2002) reported that SGA infants had a higher 1-year infant mortality rate compared with that of normally grown infants. Boulet and associates (2006) demonstrated that for a fetus at the 10th percentile, the risk of neonatal death is increased but varies with gestational age. Risk is increased threefold at 26 weeks’ gestation compared with only a 1.13-fold increased risk at 40 weeks. More recently, in an analysis of 123,383 nonanomalous singleton live births, Chen and coworkers (2011) reported that SGA infants had a twofold risk for early and late neonatal death but not for postneonatal demise.
Long-Term Sequelae
Fetal Undergrowth. In his book Fetal and Infant Origins of Adult Disease, Barker (1992) hypothesized that adult mortality and morbidity are related to fetal and infant health. This includes both under- and overgrowth. In the context of fetal-growth restriction, there are numerous reports of a relationship between suboptimal fetal nutrition and an increased risk of subsequent adult hypertension, atherosclerosis, type 2 diabetes, and metabolic derangement (Gluckman, 2008). The degree to which low birthweight mediates adult disease is controversial. For example, it is unclear whether poor health in adulthood is mainly modulated by low birthweight, postnatal compensatory growth, or an interaction of the two (Crowther, 2008; Kerkhof, 2012; Leunissen, 2008; Nobili, 2008; Ong, 2007).
There is increasing evidence that fetal-growth restriction may affect organ development, particularly that of the heart. Individuals with low birthweight demonstrate cardiac structural changes and dysfunction persisting through childhood, adolescence, and adulthood. Crispi and coworkers (2012) studied 50 children aged between 3 and 6 years who were born small-for-gestational age at > 34 weeks and compared them with 100 normally grown children. The heart shape was altered in children born SGA. They had a more globular ventricle that resulted in systolic and diastolic dysfunction. Hietalampi and associates (2012) performed echocardiography in 418 adolescents at a mean age of 15 years and found that low birthweight was associated with increased left ventricular posterior wall thickness. Importantly, current weight and physical activity affected measurements. In another study, 102 adults previously born preterm at a mean gestational age of 30.3 weeks underwent cardiac magnetic resonance (MR) imaging. Their ventricular mass was increased compared with that of 132 adults who were delivered at term (Lewandowski, 2013). Adults previously born preterm also had persistent structural remodeling and had reduced systolic and diastolic function.
Deficient fetal growth is also associated with postnatal structural and functional renal changes. Ritz and colleagues (2011) reviewed the numerous studies associating low birth-weight with disordered nephrogenesis, renal dysfunction, chronic kidney disease, and hypertension. They found that although a low nephron number is associated with hypertension in white individuals, this association does not hold true for blacks.
Fetal Overgrowth. On the other end of the spectrum, fetal overgrowth, particularly in women with diabetes and elevated cord blood levels of IGF-I, is associated with increased neonatal fat mass and morphological heart changes. Aman and coworkers (2011) reported interventricular septal hypertrophy in overgrown neonates of mothers with well-controlled diabetes in pregnancy. Garcia-Flores (2011) also reported increased fetal cardiac intraventricular septal thickness using sonography in women with well-controlled diabetes. Large-for-gestational age infants delivered of women without impaired glucose tolerance show higher insulin levels in childhood (Evagelidou, 2006). Not surprisingly, fetal overgrowth has been associated with development of the metabolic syndrome even in childhood (Boney, 2005).
Accelerated Lung Maturation
Numerous reports describe accelerated fetal pulmonary maturation in complicated pregnancies associated with growth restriction (Perelman, 1985). One possible explanation is that the fetus responds to a stressed environment by increasing adrenal glucocorticoid secretion, which leads to accelerated fetal lung maturation (Laatikainen, 1988). Although this concept pervades modern perinatal thinking, there is negligible evidence to support it.
To examine this hypothesis, Owen and associates (1990) analyzed perinatal outcomes in 178 women delivered because of hypertension. They compared these with outcomes in infants of 159 women delivered because of spontaneous preterm labor or ruptured membranes. They concluded that a “stressed” pregnancy did not confer an appreciable survival advantage. Similar findings were reported by Friedman and colleagues (1995) in women with severe preeclampsia. Two studies from Parkland Hospital also substantiate that the preterm infant accrues no apparent advantages from fetal-growth restriction (McIntire, 1999; Tyson, 1995).
Risk Factors and Etiologies
Risk factors for impaired fetal growth include potential abnormalities in the mother, fetus, and placenta. These three “compartments” are depicted in Figure 44-4. Some of these factors are known causes of fetal-growth restriction and may affect more than one compartment. For instance, infectious causes such as cytomegalovirus may affect the fetus directly. In contrast, bacterial infections such as tuberculosis may have significant maternal effects that lead to poor fetal growth. Malaria, a protozoal infection, possibly creates placental dysfunction (Umbers, 2011). Importantly, many causes of diminished fetal growth are prospectively considered risk factors, because impaired fetal growth is not consistent in all affected women.
FIGURE 44-4 Risk factors and causes of impaired fetal growth centering on the mother, her fetus, and the placenta.
Constitutionally Small Mothers
It is axiomatic that small women typically have smaller newborns. If a woman begins pregnancy weighing less than 100 pounds, the risk of delivering an SGA infant is increased at least twofold (Simpson, 1975). As discussed subsequently, both prepregnancy weight and gestational weight gain modulate this risk. Durie and colleagues (2011) showed that the risk of delivering an SGA neonate was highest among underweight women who gained less weight than recommended by the Institute of Medicine (Chap. 9, p. 177). Also, both maternal and paternal size influences birthweight. In a Swedish study of 137,538 term singleton mother-father-child units, researchers estimated that the maternal and paternal birthweights explained 6 and 3 percent of variance in birthweight, respectively (Mattsson, 2013).
Gestational Weight Gain and Nutrition
In the woman of average or low BMI, poor weight gain throughout pregnancy may be associated with fetal-growth restriction (Rode, 2007). In the study by Durie cited above, gestational weight gain during the second and third trimesters that was less than that recommended by the Institute of Medicine was associated with SGA neonates in women of all weight categories except class II or III obesity. Conversely, excessive gestational weight gain was associated with an overgrown newborn in all weight categories.
As perhaps expected, eating disorders are associated with significantly increased risks of low birthweight and preterm birth (Pasternak, 2012). This is discussed further in Chapter 61 (p. 1211). Marked weight gain restriction after midpregnancy should not be encouraged even in obese women (Chap. 9, p. 177). Even so, it appears that food restriction to < 1500 kcal/day adversely affects fetal growth minimally (Lechtig, 1975). The best documented effect of famine on fetal growth was in the Hunger Winter of 1944 in Holland. For 6 months, the German Occupation army restricted dietary intake to 500 kcal/day for civilians, including pregnant women. This resulted in an average birthweight decline of only 250 g (Stein, 1975).
It is unclear whether undernourished women may benefit from micronutrient supplementation. In the study by the Supplementation with Multiple Micronutrients Intervention Trial (SUMMIT) Study Group (2008), almost 32,000 Indonesian women were randomized to receive micronutrient supplementation or only iron and folate tablets. Infants of those receiving the supplement had lower risks of early infant mortality and low birthweight and had improved childhood motor and cognitive abilities (Prado, 2012). Conversely, Liu and coworkers (2013) randomized 18,775 nulliparous pregnant women to folic acid alone; folic acid and iron; or folic acid, iron, and 13 other micronutrients. Folic acid and iron with or without the additional micronutrients resulted in a 30-percent reduction in risk of third-trimester anemia. However, it did not affect other maternal or neonatal outcomes. The importance of antenatal vitamins and trace metals is further discussed in Chapter 9 (p. 179).
Social Deprivation
The effect of social deprivation on birthweight is interconnected to the influence of associated lifestyle factors such as smoking, alcohol or other substance abuse, and poor nutrition. Importantly, Coker and associates (2012) found that women screened during pregnancy for psychosocial risk factors had more appropriate interventions. These women were significantly less likely to deliver a low-birthweight infant and also had fewer preterm births and other pregnancy complications.
Women who are immigrants may be at particular risk in pregnancy. Poeran and colleagues (2013) studied 56,443 singleton pregnancies in Rotterdam between 2000 and 2007 and found that social deprivation was associated with adverse perinatal outcomes that included SGA infants among socially deprived women. However, a similar linkage was not noted in socially deprived women of non-Western origin. The effect of immigration, however, is complex and dependent on the population studied. A paradoxical relationship between pregnancy outcomes in foreign-born Latina women delivering in the United States compared with Latina women born in the United States has been described (Flores, 2012). In particular, foreign-born Latina women appear to have lower risks of preterm birth and SGA neonates.
Vascular Disease
Especially when complicated by superimposed preeclampsia, chronic vascular disease commonly causes growth restriction (Chap. 50, p. 1004). Preeclampsia may cause fetal-growth failure, which can be an indicator of its severity (Backes, 2011). In a study of more than 2000 women, vascular disease as evidenced by abnormal uterine artery Doppler velocimetry early in pregnancy was associated with increased rates of preeclampsia, SGA neonates, and delivery before 34 weeks (Groom, 2009). Roos-Hesselink and coworkers (2013) described pregnancy outcomes in women with heart disease, and only 25 of the 1321 women had ischemic heart disease, emphasizing its rareness. But these women had the worst outcomes, with significantly lower neonatal birthweights and the highest rates of preterm birth and perinatal mortality.
Renal Disease
Chronic renal insufficiency is frequently associated with underlying hypertension and vascular disease. Nephropathies are commonly accompanied by restricted fetal growth (Bramham, 2011; Cunningham, 1990; Vidaeff, 2008). These relationships are considered further in Chapter 53 (p. 1059).
Pregestational Diabetes
Fetal-growth restriction in women with diabetes may be related to congenital malformations or may follow substrate deprivation from advanced maternal vascular disease (Chap. 57, p. 1128). Also, the likelihood of restricted growth increases with development of nephropathy and proliferative retinopathy—especially in combination (Haeri, 2008). That said, the prevalence of serious vascular disease associated with diabetes in pregnancy is low, and the primary effect of overt diabetes, especially type 1, is fetal overgrowth. For example, Murphy and coworkers (2011) performed a prospective study of 682 consecutive pregnancies complicated by diabetes. Women with type 1 diabetes had significantly fewer of the traditional risk factors for fetal overgrowth, such as increased age, multiparity, and obesity. Yet, they were significantly more likely than women with type 2 diabetes to have a neonate weighing > 90th and 97.7th percentiles. Additionally, women with type 1 diabetes were significantly less likely to deliver an SGA newborn. Similarly, in a smaller study by Cyganek and colleagues (2011), the rate of macrosomia was higher among pregnancies complicated by type 1 diabetes. However, the rates of low birthweight were similar for those with type 1 and type 2 diabetes.
Chronic Hypoxia
Conditions associated with chronic uteroplacental hypoxia include preeclampsia, chronic hypertension, asthma, smoking, and high altitude. When exposed to a chronically hypoxic environment, some fetuses have significantly reduced birthweight. Gonzales and Tapia (2009) constructed growth charts based on 63,620 Peruvian live births between 26 and 42 weeks’ gestation. They reported that mean birthweight was significantly decreased at higher altitudes compared with lower altitudes—3065 ± 475 g versus 3280 ± 525 grams. At low altitude, the rate of birthweight < 2500 g was 6.2 percent, and it was 9.2 percent at high altitude. In contrast, the rate of birthweight > 4000 g was 6.3 percent at low altitude and 1.6 percent at high altitude. As discussed in Chapter 49 (p. 985), severe hypoxia from maternal cyanotic heart disease frequently is associated with severely growth-restricted fetuses (Patton, 1990).
Anemia
In most cases, maternal anemia does not cause restricted fetal growth. Exceptions include sickle-cell disease and some other inherited anemias (Chakravarty, 2008; Tongsong, 2009). Conversely, curtailed maternal blood-volume expansion has been linked to fetal-growth restriction (Duvekot, 1995; Scholten, 2011). This is further discussed in Chapter 40 (p. 737).
Antiphospholipid Antibody Syndrome
Adverse obstetrical outcomes including fetal-growth restriction have been associated with three species of antiphospholipid antibodies: anticardiolipin antibodies, lupus anticoagulant, and antibodies against beta-2-glycoprotein-I. Mechanistically, a “two-hit” hypothesis suggests that initial endothelial damage is then followed by intervillous placental thrombosis. More specifically, oxidative damage to certain membrane proteins such as beta-2-glycoprotein I is followed by antiphospholipid antibody binding, which leads to immune complex formation and ultimately to thrombosis (Giannakopoulos, 2013). This syndrome is considered in detail in Chapters 52 (p. 1033) and 59 (p. 1173). Pregnancy outcomes in women with these antibodies may be poor and include early-onset preeclampsia and fetal demise (Levine, 2002). The primary autoantibody that predicts obstetrical antiphospholipid syndrome appears to be lupus anticoagulant (Lockshin, 2012).
Inherited Thrombophilias
Numerous investigators have evaluated the role of genetic polymorphisms in the mother or fetus and their relationship to growth restriction (Lockwood, 2002; Stonek, 2007). Most suggest that inherited thrombophilias are not a significant factor in fetal-growth restriction (Infante-Rivard, 2002; Rodger, 2008). Facco and colleagues (2009) attribute positive associations primarily to publication bias.
Infertility
Pregnancies in women with prior infertility with or without infertility treatment have an increased risk of SGA newborns (Zhu, 2007). Kondapalli and Perales-Puchalt (2013) have recently reviewed possible links between low birthweight and infertility with its various interventions. They concluded that the association remains unexplained.
Placental and Cord Abnormalities
Several placental abnormalities may cause poor fetal growth. These are discussed further throughout Chapter 6 and include chronic placental abruption, extensive infarction, chorioangioma, marginal or velamentous cord insertion, placenta previa, and umbilical artery thrombosis. Growth failure in these cases is presumed secondary to uteroplacental insufficiency.
Abnormal placental implantation leading to endothelial dysfunction may also result in limited fetal growth (Brosens, 2011). This pathology has been implicated in pregnancies complicated by preeclampsia as discussed in Chapter 40 (p. 732).
If the placenta is implanted outside the uterus, the fetus is usually growth restricted (Chap. 19, p. 388). Also, some uterine malformations have been linked to impaired fetal growth (Chap. 3, p. 40).
Multiple Fetuses
As shown in Figure 44-5, pregnancy with two or more fetuses is more likely to be complicated by diminished growth of one or more fetuses compared with that with normal singletons (Chap. 45, p. 899).
FIGURE 44-5 Birthweight and gestational age relationships in multifetal gestations delivered at Parkland Hospital without malformations. (Data courtesy of Dr. Don McIntire.)
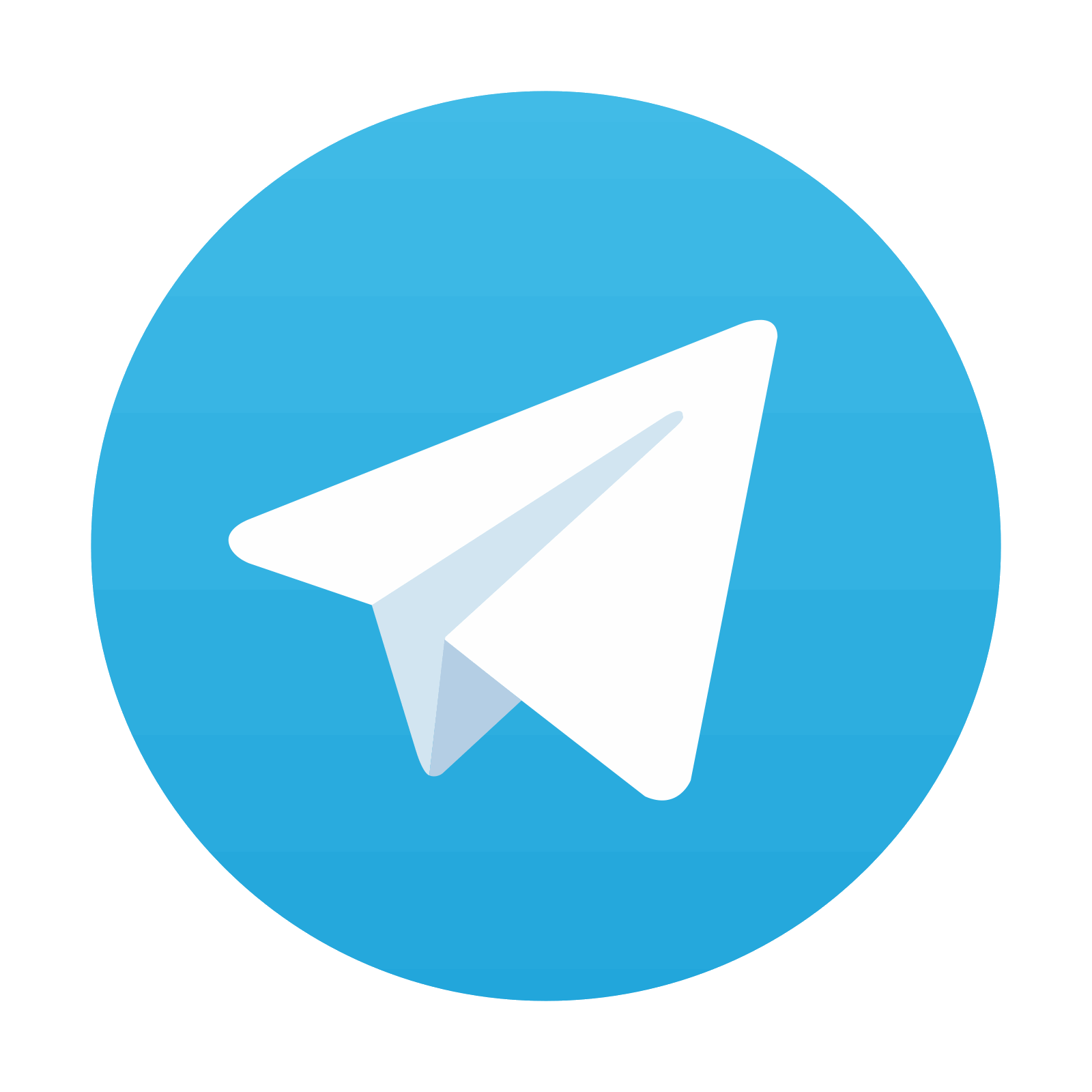
Stay updated, free articles. Join our Telegram channel
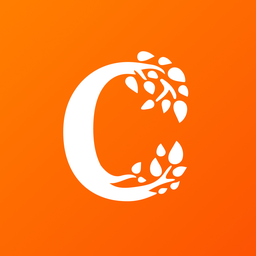
Full access? Get Clinical Tree
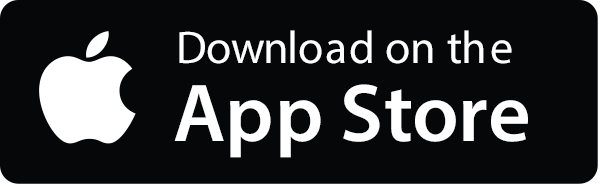
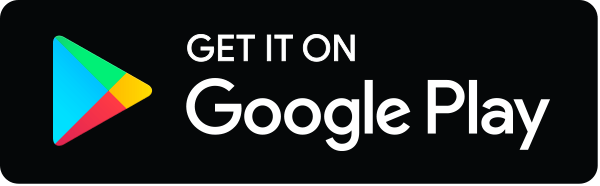