First described in the 1970s, high-frequency ventilation (HFV) is a form of mechanical ventilation that uses small tidal volumes, sometimes less than anatomic dead space, and very rapid ventilator rates (2 to 20 Hz or 120 to 1200 cycles/min). Potential advantages of this technique over conventional mechanical ventilation (CMV) include the use of lower peak airway pressures, the ability to adequately and independently manage oxygenation and ventilation while using very small tidal volumes, and the preservation of normal lung architecture even when using high mean airway pressures. HFV’s ability to sufficiently oxygenate and ventilate the fragile preterm lung with peak airway pressures that are lower than those used with CMV, as well as its use for alveolar recruitment and distribution of medications such as inhaled nitric oxide (iNO), makes HFV a crucial constituent of neonatal respiratory therapy. In this chapter, current HFV techniques and technology are described, and their application in the newborn with pulmonary dysfunction is discussed.
Currently, there are three general types of HFV: high-frequency jet ventilation (HFJV), which is produced by ventilators that deliver a high-velocity jet of gas directly into the airway and have passive exhalation; high-frequency oscillatory ventilation (HFOV), which is produced by a device that moves air back and forth at the airway opening and produces limited bulk gas flow; and high-frequency flow interruption (HFFI), which generates pulses of fresh gas and also uses passive exhalation. HFV appears to enhance both the distribution and the diffusion of respiratory gases. Both shift the transition point between convective and diffusive gas transport progressively in a cephalad direction from the acinus into the larger airways. The net effect of this shift is efficient CO 2 elimination relatively independent of mean lung volume.
Conventional pulmonary physiology tells us that the amount of gas available for gas exchange, the alveolar tidal volume (V A ), is the product of the tidal volume (V T ) delivered into the airway minus anatomic dead space (V D ): V A = V T − V D . If this relationship is true, V T s near anatomic V D should produce little if any alveolar ventilation. In an attempt to clarify the mechanisms of HFV gas exchange at V T less than anatomic V D , Chang demonstrated that multiple modes of gas transport occur, including bulk convection, high-frequency “pendelluft,” convective dispersion, Taylor-type dispersion, and molecular diffusion. Whatever the HFV system, the presumed linear relationship between ventilator rate and CO 2 elimination is no longer valid with this mode of ventilation. In fact, during HFOV, CO 2 elimination improves with decreasing ventilator frequency as long as the inspiratory-to-expiratory time ratio (I:E) is held constant. This is because a reduction in frequency produces increased V T and minute volume out of proportion to the change, whereas an increased frequency does the opposite. The effects are magnified because minute ventilation is roughly equal to <SPAN role=presentation tabIndex=0 id=MathJax-Element-1-Frame class=MathJax style="POSITION: relative" data-mathml='f×VT2′>f×V2Tf×V2T f×VT2 f×V2T f × V T 2 . The same could be true with HFJV, but with that device the inspiratory time is normally kept at the shortest value, and thus the I:E ratio decreases as frequency goes down and therefore the V T remains the same.
Although paradoxical at first blush, this inverse relationship reflects the impact of an increasing inspiratory time as frequency is lowered, which results in higher delivered gas volumes. In 1980, Slutsky et al. reported a review of possible gas transport mechanisms during HFV. They suggested that CO 2 elimination during HFV varies according to the product of frequency a and <SPAN role=presentation tabIndex=0 id=MathJax-Element-2-Frame class=MathJax style="POSITION: relative" data-mathml='VTb’>VbTVbT VTb VbT V T b , with b greater than 1 and a less than 1. Since then, many other theoretical and practical reviews have been published, and we know that during HFV carbon dioxide removal is proportional to f a × <SPAN role=presentation tabIndex=0 id=MathJax-Element-3-Frame class=MathJax style="POSITION: relative" data-mathml='VTb’>VbTVbT VTb VbT V T b , where a < 1 and b > 1. In 2002, Slutsky and Drazen concisely summarized these gas exchange mechanisms ( Fig. 22-1 ). The theoretical mechanisms of gas exchange during HFV are beyond the scope of this clinical chapter. For those interested, there are a number of excellent review articles, as well as a review of physiologic principles in Chapter 2 .

Types of High-Frequency Ventilators
As of this writing, only a small number of HFV devices are approved by the U.S. Food and Drug Administration (FDA) for clinical use. Even though the number of high-frequency ventilators is small, the classification or taxonomy of these ventilators is confusing and at times inconsistent. Froese and Bryan classified HFV into three categories based on the character of exhalation: active, passive, and hybrid. In this chapter we use the more traditional, clinical classification of HFFI, HFJV, and HFOV. Table 22-1 lists all the approved HFV devices in the United States and Canada and their classification. A number of other HFV devices have been studied and are currently being used throughout the world. This chapter focuses primarily on those machines currently approved for clinical use in the United States and Canada.
Ventilator | HFV Type | CV + HFV | Volume Measurement | Exhalation |
---|---|---|---|---|
SensorMedics 3100A | Diaphragm oscillator | No | No | Active |
Dräger V500, VN500 | Diaphragm oscillator | Yes | Yes | Active |
Fabian HFO | Diaphragm oscillator | Yes | Yes | Active |
Leoni Plus | Diaphragm oscillator | Yes | Yes | Active |
SLE5000 | Reverse jet oscillator | Yes | Yes | Active |
VDR-4/Bronchotron | Flow interrupter | Yes | No | Passive |
Bunnell Life Pulse | Jet pulse | No | No | Passive |
Determinants of Gas Transport during Mechanical Ventilation
During conventional inflation, the time required for gas to travel from one end of the airway to the other depends on a ventilator’s peak inflation pressure (PIP), inspiratory gas flow, and pressure–flow waveforms (compliance of the respiratory system and resistance of the airways). During expiration, the time required for lung emptying depends mainly on lung and chest wall elastic recoil, expiratory resistance, and the ventilator’s set positive end-expiratory pressure (PEEP). If inspirations or expirations are long enough, proximal and distal airway pressures equilibrate and gas flow stops. Conditions are static at end inspiration and end expiration. The volume of gas delivered into or out of the lung is determined by the pressure change in the lung and lung compliance (Δ V = Δ P × CL ). As ventilator rates increase, inspiratory and expiratory times decrease. Eventually, there is inadequate time for proximal and distal airway pressures to equilibrate. Gas flow is continuous. Conditions are no longer static. They are dynamic. The volume of gas delivered into or out of the lung becomes a function of flow rate and time.
The key timing factors of gas transport depend upon lung compliance and airway resistance. The product of lung compliance and airway resistance, measured in seconds, is called its time constant (see Chapter 2 ). Time constants describe the time it takes for proximal and terminal airway pressures to equilibrate. After one time constant, there is 63% equilibration; after two, 84.5%; after three, 95%. After five time constants, there is 99% pressure equilibration between the proximal and the terminal airways. When inspiratory time is shortened beyond three time constants, a pressure gradient develops between the proximal and the terminal airways. As inspiratory time becomes shorter, this pressure gradient progressively increases. Because the V T delivered to the terminal airways is also a function of this gradient, it too becomes a function of inspiratory time. As the pressure gradient between the proximal and the terminal airways increases, the volume of gas delivered into the terminal airways decreases in a predictable way.
Time constants apply equally to inspiration and expiration. Because airway resistance is always greater during expiration, expiratory time constants are always longer. Experimental evidence suggests that this difference between inspiratory and expiratory resistances increases as ventilator rates increase. Expiratory times less than three time constants do not allow adequate lung emptying and ultimately result in increasing functional residual capacity levels and gas trapping. Because of these factors, inadequate gas delivery during inflation and incomplete lung emptying during expiration limit the effectiveness of gas exchange when using high rates during conventional ventilation (high-frequency positive pressure ventilation, HFPPV).
One can assess for these limiting factors, because today it is relatively easy to estimate respiratory mechanics and the impact of ventilator manipulation at the bedside. Most conventional neonatal ventilators now display measurements of V T , minute ventilation, airway pressures, and respiratory cycle timing, as well as basic measurements of respiratory mechanics. The simultaneous tracings of proximal airway pressures, gas flows, and volume delivery show whether time constants are violated and create a potential environment for suboptimal gas exchange and/or gas trapping. For example, when gas flow patterns during inflation reach a plateau, proximal and distal airway equilibration has occurred, and prolonging the inspiratory time beyond this point serves only to increase airway pressures. Conversely, decreasing inspiratory time below this point decreases delivered V T and probably would lead to suboptimal ventilation. Expiratory flow patterns provide similar information regarding lung emptying and gas trapping.
High-Frequency Jet Ventilators
High-frequency jet ventilators deliver short pulses of pressurized gas directly into the upper airway through a narrow-bore cannula or jet injector. High-frequency jet ventilators are capable of maintaining ventilation over wide ranges of patient sizes and lung compliances. These systems have negligible compressible volumes. The only jet device approved for neonatal use in the United States and Canada is the Bunnell jet ventilator; it operates at rates from 240 to 600 cycles/min (4 to 10 Hz), with the most common rates, 240 to 420 cycles/min, being less than those typically used in HFOV. Exhalation during HFJV is a result of passive lung recoil. An open ventilator–patient circuit is essential, and HFJV is used in combination with a CMV to provide PEEP and occasional sigh breaths if needed. V T s are difficult to measure but appear to be equal to or slightly greater than anatomic V D .
In addition to V T s delivered through the jet injector, gases surrounding the injector are pulled or entrained into the airway with each jet pulse. The high-flow jet pulse produces a Venturi effect that creates an area of negative pressure at its periphery, entraining ambient gases into the airway. Because of high gas velocities, Venturi effects, and pressure gradients within the delivery system, pressure monitoring is difficult with HFJV. Airway pressures must be measured far enough downstream from the jet injector to minimize Venturi effects. Pressures measured upstream from the jet injector are meaningless unless such effects have been accounted for. Jet ventilation using the Bunnell device is administered via a triple-lumen endotracheal tube adapter. This adapter houses the jet injector port and the pressure monitoring port.
Jet ventilators have been tested extensively in laboratory animals and have been used clinically in adults and neonates for over 30 years. The Bunnell Life Pulse jet ventilator (Bunnell, Inc., Salt Lake City, Utah) was designed specifically for infants ( Fig. 22-2 ). Using the triple-lumen endotracheal tube adapter, this device delivers its jet pulse into the endotracheal tube through the adapter’s injector port and then servo controls the background pressure, or driving pressure, of the jet pulse to maintain a constant user preset pressure within the endotracheal tube. This device is approved for clinical use in neonates and infants. With HFJV, CO 2 removal can be achieved at lower peak and mean airway pressures than with either HFPPV or HFOV. Although initially primarily used to treat air leak, HFJV is also effective in homogeneous lung disorders such as respiratory distress syndrome (RDS), as a randomized multicenter trial has demonstrated a beneficial pulmonary effect (lower rates of chronic lung disease) with the use of early HFJV over CMV in RDS.

HFJV appears to be uniquely effective in nonhomogeneous lung disorders in which CO 2 elimination is the major problem, such as air-leak syndromes (i.e., pulmonary interstitial emphysema [PIE]), or in diseases in which atelectasis and overdistention occur simultaneously. In the former, the long expiratory time and the high-flow jet pulse contribute to the resolution of air leak. In the latter, the ability to provide a low-rate (2 to 6 inflations/min) sigh assists with opening atelectatic areas. It is also safe and effective when used in neonatal transport and can be used with simultaneous delivery of iNO.
In Europe the Monsoon jet ventilator (Acutronic Medical Systems AG, Hirzel, Switzerland) has been used primarily for ear, nose and throat (ENT) surgery and thoracic surgery and has seen limited applications in intensive care for patients with severe respiratory failure and air-leak complications. The device has an integrated humidification and heating system, making it potentially suitable for extended use. It operates at frequencies of 0.2 to 10 Hz, inspiratory time of 20% to 70%, PEEP 10 to 40. No systematic evaluation of safety and effectiveness is available.
High-Frequency Oscillators
High-frequency oscillators (HFOs) are a type of HFV operating at frequencies ranging from 180 to 1200 cycles/min (3 to 20 Hz) to move small volumes of gas in and out of the lungs. During HFOV, inspiration and expiration are both active (proximal airway pressures are negative during expiration). In the CareFusion (formerly SensorMedics) 3100A (CareFusion, San Diego, Calif.) a continuous flow of fresh gas flows past the electromagnetically operated piston, generating the oscillations. This bias gas flow is the system’s only source of fresh gas. A controlled-leak or low-pass filter allows gas to exit the system ( Fig. 22-3 ). The amplitude of the pressure oscillations within the airway determines the V T s that are delivered to the lungs around a constant mean airway pressure. This allows avoidance of high peak airway pressures for ventilation as well as maintenance of lung recruitment by avoidance of low end-expiratory pressures. Though piston pumps or vibrating diaphragms are often used to provide HFOV, newer HFOV devices available in Canada and Europe have a series of Venturi valves that provide effective active expiration and can also measure and control V T .

As with HFJV, pressure monitoring in HFOV is a problem. During HFOV, airway pressures usually are measured either at the proximal end of the endotracheal tube or within the ventilator itself. Many practitioners question the clinical relevance of such measurements, as they are some distance away from the patient; the relationship of intrapulmonary pressures measured during HFOV to those measured during CMV is difficult to assess accurately. Depending upon the size and resonant frequency of the lung, alveolar pressures can be the same, lower, or even higher than those measured in the trachea.
HFOs have been tested extensively in animals and in humans. Today the neonatal HFO used in the United States is the CareFusion (formerly SensorMedics) 3100A oscillator (CareFusion, San Diego, Calif.). This ventilator has been approved for clinical use in neonates and does not require a tandem conventional ventilator. This device produces its oscillations via an electromagnetically controlled piston/diaphragm. Frequency (3 to 15 Hz or 180 to 900 cycles/min), percentage inspiratory time, and volume displacement can be adjusted, as well as resistance at the end of the bias flow circuit ( Fig. 22-4 ). Variations in bias flow rate and the patient circuit outflow resistor control mean airway pressures. Ventilation (CO 2 elimination) is proportional to the product of frequency and the square of the V T ( f × <SPAN role=presentation tabIndex=0 id=MathJax-Element-4-Frame class=MathJax style="POSITION: relative" data-mathml='VT2′>V2TV2T VT2 V2T V T 2 ), and thus a decrease in frequency or increase in V T by way of an increase in set amplitude should cause increased carbon dioxide removal. The 3100B is a more powerful oscillator that can be used in children and adults. There are many other HFOs that are not currently used in the United States. These include the SLE 5000 (SLE UK Ltd.), the Fabian (Acutronic Medical Systems), the Leoni Plus (Heinen & Lowenstein), the Stephanie and the Sophie (Stephan), the VN500 (Dräger), and the BabyLog 8000+ (Dräger). The Fabian ( Fig. 22-5 ), Leoni Plus ( Fig. 22-6 ), and Dräger devices ( Fig. 22-7 ) are approved for use in Canada. A variety of mechanisms provide HFOV with these ventilators, but they are all true oscillators based on the waveform they generate.




High-Frequency Flow Interrupters
The term flow interrupter originally was used to describe a group of ventilators that were neither true oscillators nor true jets. Some had jet-type injectors but delivered their bursts of gas not directly into the airway but into the ventilator circuit some distance back from the trachea and endotracheal tube. For this reason, these machines also were called setback jets .
The Infant Star HFV (Nellcor Puritan Bennett, Pleasanton, Calif.), which is no longer in production, was once the most widely used flow interrupter. This ventilator had a set of microprocessor-controlled pneumatic valves that altered inspiratory flow to achieve preset PIPs. Although there was a Venturi system on the exhalation valve to facilitate expiration and prevent inadvertent PEEP, exhalation was still largely passive, and air trapping with air leak appeared to be a problem.
Thome and colleagues compared high-frequency flow interrupter (HFFI) ventilation using the Infant Star HFV system to rapid-rate conventional ventilation with several different neonatal ventilators. The outcomes showed no benefit of HFFI ventilation, and babies treated with the Infant Star HFFI had significantly more air leaks. In the “Sy-Fi” study, Craft and colleagues again compared the Infant Star system to CMV in extremely low birth-weight infants and found no difference in air leaks or other pulmonary outcomes with a nonsignificant trend to more air leak with the Infant Star (36% vs 25%).
In recent years, HFFI ventilation has experienced a modest resurgence in the form of several devices from the company of Forrest Bird, the inventor of the first neonatal ventilator, the BabyBird ® (Percussionaire Corp., Sagle, Idaho). The Bronchotron ® is a pneumatically driven flow interrupter/percussive ventilator increasingly used during transport.
The Bronchotron ® is attractive as a transport ventilator because of its light weight, ability to function as both conventional and high-frequency ventilator, and relatively low gas consumption. The ventilator’s internal pneumatic timer cycles high-pressure gas flow at a frequency ranging from 3 to 10 Hz. Rate and amplitude are continuously adjustable. The inspiratory time is determined by the frequency and the mechanical properties of the lungs. The high-frequency gas pulses enter a sliding piston mechanism called a Phasitron ® through a Venturi cavity in its central axis. The Phasitron ® creates pulses of gas flow by the rapid movement of a spring mechanism that balances inspiratory and expiratory pressures within preset pressures for PEEP or <SPAN role=presentation tabIndex=0 id=MathJax-Element-5-Frame class=MathJax style="POSITION: relative" data-mathml='P¯aw’>P⎯⎯⎯awˉPaw P¯aw ˉPaw P ¯ aw , acting as both an inspiratory and an expiratory valve. In the inspiratory phase, the pulse of gas is augmented by entrained gas proportional to the pressure difference before and after the Venturi. During expiration, the piston springs back, opening an exhalation port, and gas is allowed to exit the patient through an adjustable resistor that provides PEEP and regulates mean airway pressure. The mean airway pressure, frequency, and flow (which adjusts the gas flow to the Phasitron ® and controls the pulse amplitude) are continuously adjustable.
The main limitation of the device is the lack of real values for the ventilator variables—all dials are marked with values of 1 to 10, but these numbers do not readily translate to values to which a clinician can relate. Adjustments must be made based solely on clinical observation of chest movement and patient response. Frequency is displayed as cycles/min (not Hz), and mean airway pressure can be measured intermittently by flipping a toggle switch and changing the phasic pressure display to an integrated mean. The phasic pressure displayed by the rapidly oscillating needle of a mechanical gauge is difficult to read. The other major concern is a lack of alarms or other safety features.
The safety and efficacy of the Bronchotron ® are not well documented; the device was “grandfathered” by the FDA, meaning that it was approved based on its “substantial equivalence” to a device in existence prior to the effective date of the law (1979). In a preclinical study in saline-lavaged newborn piglets the Bronchotron ® and the 3100A oscillator achieved similar gas exchange when the devices were adjusted to deliver identical V T at the same mean airway pressure and frequency, but a higher pressure amplitude was needed with the Bronchotron ® .
The VDR-4 ® (Percussionaire Corp., Sagle, Idaho) is a time-cycled, pressure-controlled, pneumatically driven high-frequency percussive ventilator similar to the Bronchotron ® but more complex and designed for hospital use. The device delivers gas from a pressurized source through a pneumatic timing cartridge system. The source gas is interrupted to produce a pulsatile flow, which enters the breathing circuit via the Phasitron ® as with its sister device. Warmed, humidified gas is entrained to augment V T . V T delivery is determined by flow velocity, inspiratory duration, and supplementary gas entrainment. The VDR-4 ® is composed of two subsystems: conventional and high frequency. The conventional component can deliver up to 70 inflations per minute with independent control of inflation time and pressure. The high-frequency component can deliver frequencies from 0.5 to 30 Hz, amplitudes from 0 to 100 cm H 2 O, and inspiratory to expiratory time ratio from 1:1 to 1:5. A variety of conventional and high-frequency combinations can be used. Like the Bronchotron ® , the VDR was approved by the FDA without requiring proof of safety and efficacy. The literature regarding the safety and efficacy of this device is limited to a few small studies in adult and pediatric patients and a single case series of six newborn infants. There is also some interest in using this device to deliver HFV vial the nasal route.
Because all conventional pressure-preset neonatal ventilators will generate rates up to 150 inflations/min, theoretically all of them can be used to produce rapid-rate conventional ventilation, sometimes referred to as HFPPV. Use of a conventional ventilator at these rates is now mostly of historical interest. The term HFPPV most often refers to mechanical ventilators operating at rates between 60 and 150 inflations/min (1 to 2.5 Hz). Sjostrand pioneered this technique in Sweden using specially designed ventilators with extremely low compressible volumes. He and his colleagues studied more than 2000 adults and children during surgery and 32 neonates with RDS. He concluded that in most clinical situations, HFPPV provided adequate respiratory support. In 1980, Bland et al. reported improved outcomes in 24 infants with RDS using conventional volume-preset infant ventilators operating at rates ranging from 60 to 110 inflations/min. In 1991, a multicenter randomized trial compared HFPPV using rates of 60 inflations/min to CMV using rates up to 40 inflations/min. The infants treated with HFPPV had fewer pulmonary air leaks. Few people today would consider a rate of 60 inflations/min in the neonate as HFV.
Though CMVs can operate at rates up to 150 inflations/min, few of these machines were designed with such frequencies in mind. In vitro and in vivo studies of conventional pressure-preset infant ventilators show that all have maximum operating frequencies beyond which their performance deteriorates and inadvertent PEEP becomes a problem.
The first such in vitro study measured delivered tidal and minute volumes as ventilator rates increased progressively from 20 to 150 inflations/min. All the machines tested had maximal effective rates beyond which minute ventilation decreased exponentially. For the compliance and resistance values studied, these maximum effective rates ranged from 75 to 100 inflations/min ( Fig. 22-8, A ). A subsequent animal study showed remarkably similar results. This study also noted that as CMV rates increased and minute ventilation decreased, functional residual capacities progressively increased. Hird et al. studied human neonates and confirmed such gas trapping at higher rates as well, in particular in paralyzed infants. Fontan et al. studied rabbits and saw predictably reduced compliance and V T values at higher rates.

When in vitro studies of ventilator performance were repeated using currently available neonatal ventilators, performance was more consistent as rates increased, but marked intradevice variability was demonstrated at similar pressures ( Fig. 22-8, B ). These studies all suggest that CMVs cycling at the upper limits of their frequency range to produce HFPPV require higher, not lower, airway pressures to maintain adequate gas exchange. Thus, some other form of HFV is much more commonly employed today, but as of this writing, in the United States, this requires changing to a different device. In Europe and Canada, ventilators that switch from conventional ventilation (CV) to HFOV are easily available.
Clinical Applications of High-Frequency Ventilation
Elective versus Rescue High-Frequency Ventilation
HFV has been studied in animal models for over 30 years. The majority of animal data supports the superiority of HFV over CMV, in terms of both short-term physiology and pressure exposure and in lung pathology over days to weeks. Animal studies suggest that HFV works at lower proximal airway pressures than CMV, reduces ventilator-induced lung injury and lung inflammatory markers, improves gas exchange in the face of air-leak syndromes, is synergistic with surfactant, and decreases oxygen exposure. Unfortunately, these findings have not been consistently reproduced in the human studies of HFV versus CMV, when looking at HFV either as an initial, elective mode of ventilation or as a rescue mode of ventilation when CMV has failed to provide adequate gas exchange.
As of this writing there have been 16 randomized controlled trials (RCTs) of elective use of HFV versus CMV for the treatment of neonates with respiratory insufficiency, primarily in babies with RDS of prematurity. The studies include HFV in the forms of HFPPV, HFFI, HFJV, and HFOV. The majority of the studies (11 of 16) were unable to demonstrate any significant difference in pulmonary outcomes between babies treated with HFV versus CMV. The remainder of the studies demonstrated a small yet significant reduction in bronchopulmonary dysplasia (BPD) in the HFV-treated groups. In 2009, the Cochrane database provided a review and meta-analysis of clinical trials of elective HFOV versus CMV in preterm infants with acute pulmonary dysfunction. The review demonstrated no evidence of effect on mortality and no clear advantage to the preferential use of elective HFO over CMV as the initial ventilation strategy in premature babies with respiratory distress. An individual patient data meta-analysis confirmed these results.
An “optimal lung volume” strategy with HFOV, piston oscillators, lack of lung-protective strategies in the CMV groups, early use of HFO (less than 6 hours), I:E of 1:2, and extubation to continuous positive airway pressure rather than a trial of CV were associated with the trials that demonstrated a reduction in chronic lung disease in the HFO groups. The Cochrane database also reviewed the elective use of HFJV versus CMV and from the three studies reviewed concluded that there may be a decreased risk of BPD in the elective HFJV groups. However, the authors raised questions about these apparent positive findings because of significant heterogeneity among the studies and the fact that one study showed increased adverse neurologic outcomes in the HFJV group. This finding appears to be related to the significant hypocarbia seen in the HFJV group and was also apparent in a subgroup analysis of the larger multicenter trial. The fact that two studies of HFV using the same device in a virtually identical population obtained very different results highlights the importance of using an appropriate ventilation strategy. Overall, grouped analysis of all randomized, controlled studies to date would not support the selective use of early and elective HFV over CMV in premature babies with respiratory insufficiency. This is very likely due to the improved methods of CV in use today, including volume-targeted ventilation.
One of the early HFV versus CMV RCTs, the HIFI study, raised concerns, as the HFOV-treated group demonstrated increased incidence of intraventricular hemorrhage (IVH) and/or periventricular leukomalacia (PVL). More alarming, the neurodevelopmental outcomes at 16 to 24 months postterm age were significantly worse in the HFO-treated group. Two subsequent large, multicenter, randomized trials by Courtney et al. and Johnson et al. demonstrated no difference in the rates of IVH or PVL between the HFOV- and the CMV-treated groups nor did the individual patient meta-analysis by Cool et al. Studies by Truffert et al. and Marlow et al. have now provided long-term neurodevelopmental follow-up at 2 years of age from their initial RCTs of HFOV versus CMV in preterm babies with RDS. Both studies concluded that elective use of HFOV does not portend any worsening of the long-term neurologic status. Truffert and colleagues state that early use of HFOV may in fact be associated with a better neuromotor outcome at 2 years of age. Zivanovic et al. found superior lung function at 11 to 14 years of age in the infants from the Johnson et al. trial. Although valuable, more follow-up data are necessary before widespread generalizations can be made regarding predicted long-term outcomes.
So, if elective use of HFV has not demonstrated any clear advantage over CMV for RDS, what about the use of rescue HFV when CMV appears to be failing to provide adequate gas exchange? To date, there are four RCTs in premature and term infants assessing HFV as a rescue technique after failing CMV. The data set is limited and includes two studies using HFJV and two studies using HFOV. In a first trial treating premature infants, the HIFO trial demonstrated improved gas exchange and lower rate of new air leak with HFOV. There was no effect on existing air leak and no difference in overall pulmonary outcomes and a marginally increased rate of severe IVH. Keszler’s trial, which specifically enrolled infants with PIE, showed improvement of PIE in the HFJV group versus those who remained on CMV. In the two trials treating older preterm babies (more than 34 weeks), there was notable improvement in gas exchange and treatment success in the HFV groups; however, there was no significant difference in the incidence of BPD or death between those rescued with HFV and those who remained on CMV. A meta-analysis of rescue HFV versus CMV in the Cochrane database demonstrates that there is no long-term benefit conferred on the patient by using rescue HFOV or HFJV over continued CMV. Whether lumping results from HFV studies using different modes of HFV in different populations together is appropriate is a debatable point. The HFJV study did, in fact, demonstrate improved survival attributable to HFJV when the effect of crossover was taken into account. Of note, most of these HFV rescue trials were performed when the administration of exogenous surfactant and maternal antenatal steroids were not routine standard of care. More importantly, there have been no long-term neurodevelopmental or pulmonary outcome follow-up data published from these rescue trials. Therefore, use of rescue HFV over continued CMV is not necessarily the better option; as always, each clinical situation will require careful consideration of the treatment possibilities from each mode of therapy.
Some circumstances may respond to the use of HFV more than others. Persistent pulmonary hypertension requiring nitric oxide therapy, with or without meconium aspiration or other lung disease, often responds best when HFOV is used for support. Similarly, postsurgical support for infants with gastroschisis, omphalocele, necrotizing enterocolitis, and other similar problems may benefit from HFV therapy in the face of lung disease and increased intra-abdominal pressures. Finally, potentially viable infants with pulmonary hypoplasia from different causes often require prolonged ventilator support, with the ongoing risk of acute pulmonary air-leak syndromes. HFV for these babies may help provide the bridge needed for growth and clinical assessment.
Lung Protective Strategies with HFV: Limiting Pressure While Optimizing Volume
Much progress has been made in the treatment of neonatal respiratory failure over the past few decades. In particular, antenatal steroids and exogenous surfactant replacement have decreased neonatal mortality and morbidity in premature infants. However, lung injury and pulmonary morbidities secondary to mechanical ventilation remain an ongoing problem in the care of premature infants. Of most concern, chronic lung disease in the form of BPD, defined as a persistent oxygen need at 36 weeks, postmenstrual age, develops in 40% of 22- to 29-week infants who survive to 36 weeks, with increasing frequency and severity as gestational age decreases. Clearly, dilemmas remain regarding optimization of both timing and mode of mechanical ventilation to decrease neonatal pulmonary morbidities. HFV has been explored aggressively as a potential ventilation strategy that would avoid the large V T s (volutrauma) and repetitive shear stress of the expansion and collapse with each CMV inflation (atelectrauma) that contributes to the development of BPD.
Although the body of literature comparing HFV and CMV is sizeable, it is difficult to compare one study to another because there is significant variation in ventilation strategies. In particular, when meta-analyses are performed, the heterogeneity among RCTs becomes obvious. The meta-analysis performed by Bollen and colleagues demonstrated that variation in ventilation strategies in both the HFV and the CMV groups most likely explains the observed differences in outcomes compared with other variables. These findings lead to key questions: Would the outcomes of these studies be different if all had employed similar ventilation strategies, and what would be the most appropriate HFV and CMV ventilation strategies? The search for the optimal lung-protective strategy is ongoing.
Animal models have shown that low V T and increased PEEP during CMV will lessen ventilator-induced lung injury (VILI). With both HFV and CV, animal studies have demonstrated that recruiting the lung to ensure open and stable alveoli can attenuate VILI. Reducing V T to avoid VILI will prevent the most important cause of VILI, volutrauma. Modestly increasing P co 2 (45 to 55 torr) to further decrease volutrauma and barotrauma has been termed permissive hypercarbia . The approach of recruiting and stabilizing the open alveoli has been termed optimal lung volume or open lung strategy. Taken together, these two concepts have been termed lung-protective ventilation . However, there is much debate as to how to actually employ lung-protective ventilation strategies at an infant’s bedside, because there are no strict criteria or guidelines and some of the guiding data are from adult literature. Nonetheless, most studies and reviews refer to an “open lung” strategy when there is a predefined FiO 2 target of 0.25 to 0.30 being used as a surrogate for optimal lung recruitment. To that end, V T s of less than 7 mL/kg with high ventilator rates and “permissive hypercarbia” on laboratory evaluation are generally considered to be “lung protective” with the goal to limit lung volume and alveolar overdistention (see Chapter 13 , Chapter 15 ).
HFV would appear to be ideal for a lung-protective strategy because it delivers very small V T s at low airway pressures while maintaining lung recruitment around a constant mean airway pressure. However, randomized clinical trials as of this writing do not consistently demonstrate benefits of HFV over CMV in terms of long-term morbidities and mortality. These findings, or lack thereof, have been ascribed to the inconsistent, and at times poorly defined, ventilation strategies in the HFV and/or CMV arm. Although we have many ventilator tools, there are few neonatal studies to guide us down one “best path” for optimum treatment. However, two meta-analyses, as well as a Cochrane database review, have concluded that volume-targeted CV decreases BPD, hypocarbia, pneumothorax, PVL, and grade 3/4 IVH. Still, ventilation strategies vary greatly from neonatal intensive care unit (NICU) to NICU across North America and the world. As pointed out by van Kaam and Rimensberger, there is much left to learn, including whether recruitment maneuvers and higher levels of PEEP could further optimize low V T CMV and whether the use of HFV over CMV can demonstrate decreased morbidity and mortality if the same lung-protective strategy is used in both groups. It is becoming increasingly apparent that lung recruitment is necessary for lung protection and that PEEP should be used as needed in different disease states to stabilize lung volume. Whether HFV will be shown to be generally superior to CV when similar strategies are used remains to be seen. However, it appears that lung volume recruitment may be easier to accomplish with HFV, at least psychologically, because there seems to be less resistance to using higher mean airway pressure with HFOV than to increasing PEEP with CV.
Applications of High-Frequency Ventilation in Specific Diseases
Respiratory Distress Syndrome
RDS continues to be the primary form of respiratory failure requiring treatment with mechanical ventilation in neonates. Treatment of acute RDS is based on principles of lung volume recruitment and optimization and is described in detail below under “Clinical Guidelines.”
Air-Leak Syndromes
Today HFV is generally accepted as a safe and effective treatment for severe pulmonary air leaks. This application was one of the original clinical uses of HFV, sometimes with dramatic results ( Fig. 22-9 ). There are very few RCTs evaluating the management of air-leak syndromes with HFV versus CMV. A British trial compared the incidences of pulmonary air leaks in 346 neonates treated with either HFPPV or CMV. Twenty-six percent of the infants treated with CMV developed air leaks compared to 19% of those who received HFPPV. Mortalities, durations of ventilation, and incidences of chronic lung disease (CLD) and IVH were similar. Keszler et al. compared HFPPV and HFJV in 144 infants with severe PIE. Sixty-one percent of those treated with HFJV improved, compared to only 37% treated with HFPPV. Forty-five percent of those who did not respond to HFPPV and were transferred to HFJV improved, whereas only 9% of the infants who did not respond to HFJV and were transferred to HFPPV improved. In addition, HFJV appeared to ventilate patients using lower proximal airway pressures. In another multicenter HFOV RDS trial (the HIFO study), the effect of HFOV on the treatment of air leak was examined. Air leaks, either PIE or pneumothorax, were present in 26 (30%) of 86 patients randomized to HFOV and in 22 (24%) of 90 patients randomized to CMV. Although a low-pressure strategy might be presumed in a study of this type, HFOV patients still required higher airway pressures for gas exchange. Air leaks occurred in 42% of HFOV patients who entered the study without air leak, compared to 63% of CMV patients ( p < 0.05). Although HFOV patients who entered the study with air leaks tended to do better than their counterparts treated with CMV, the differences were not significant.

Of all the forms of HFV considered thus far, HFJV has been the most successful with respect to the incidence and treatment of air-leak syndromes. All things considered, most forms of HFV appear to lessen the incidence of ventilator-associated pulmonary air leaks, whereas the data for the benefit for preexisting pulmonary air leaks are more convincing for HFJV. The question remains, why do pulmonary air leaks improve during HFV? One theory is that HFV produces smaller pressure fluxes within the distal airways. Pressures in the upper airway equilibrate and gas is delivered distally at a more constant distending pressure. Pressure differentials between airway and intrapleural space lessen. There is less stretching of the injured tissue. Less gas escapes during peak inflation and there are greater opportunities for self-repair. HFJV may be particularly good for air leaks because of the short inspiratory time (0.02 seconds), with relatively long expiratory time, and the extremely high accelerating inspiratory flow. An air leak will persist when gas is delivered at a pressure that opens the injured tissue, creating a low-resistance path for flow. The leak will continue during an inspiration for as long as the pressure exceeds that needed to stent the leak open. During CV or HFOV, because of both the inspiratory time and the characteristics of gas flow, a leak may persist, whereas during HFJV, it may rapidly close.
The low occurrence rates of bronchopleural and tracheoesophageal fistulas in neonates preclude the ability to perform adequate randomized clinical trials of management with HFV versus CMV. However, a few studies have formally evaluated the amount of air leak through these types of fistulas using HFV versus CMV. In the management of infants with bronchopleural fistula, Gonzales and colleagues showed a decrease in chest tube air leak when using HFJV versus CMV. Goldberg et al. and Donn et al. report similar experiences in managing infants with tracheoesophageal fistulas with HFJV. Furthermore, case reports, such as that by Bloom et al., and animal studies, such as that by Orlando et al., relay findings of an observed benefit to the use of HFV in the ventilatory stabilization of patients with tracheoesophageal or bronchopleural fistula. Although these findings are positive, the lack of RCTs makes it difficult to provide an evidence-based recommendation for the use of HFV over CMV in the treatment of bronchopleural or tracheoesophageal fistulas. As such an evidence base may never exist, the evidence available does support a trial of these therapies in these difficult conditions when more conventional approaches are failing.
Pulmonary Hypoplasia, Persistent Pulmonary Hypertension, and Inhaled Nitric Oxide
Infants with various forms of pulmonary hypoplasia may derive at least some short-term benefit from HFV. Some of these infants have associated, equally lethal, abnormalities. In such situations, HFV may provide a brief respite for diagnostic studies to identify potential survivors or confirm diagnoses for family members. In infants with congenital diaphragmatic hernia (CDH), HFV may be a useful “bridge” therapy to cannulation for extracorporeal membrane oxygenation (ECMO), although iNO has not been shown to significantly change outcomes. In the hypoplastic lung, because the number of gas-exchanging units is small, it is only logical to assume that ventilation at rapid rates using low V T s would be most effective. Because of the variety of conditions associated with pulmonary hypoplasia and their relative rarity, controlled studies are difficult to design or perform, and clear evidence-based guidelines simply are not available.
Infants with pulmonary hypoplasia associated with CDH may derive some benefit from HFV. To date there are no published controlled studies, only clinical anecdotes. There are many early case reports of infants with pulmonary hypoplasia associated with CDH treated with HFV. Most of the patients improved initially but had poor long-term outcomes if the hypoplasia was severe. In most of the patients, arterial blood gas measurements improved at lower proximal airway pressures; however, few patients survived. These reports predated ECMO.
Carter et al. studied 50 infants referred for ECMO who were first treated with HFOV. Forty-six percent improved and did not require ECMO. Four infants had pulmonary hypoplasia associated with CDH. None responded positively to HFOV. All required ECMO. Baumgart et al. reviewed results of 73 neonatal ECMO candidates who first were treated with HFJV. Nine infants had pulmonary hypoplasia associated with CDH; only three survived. deLemos et al. reviewed the outcomes of 122 neonatal ECMO candidates first treated with HFO. Fifty-three percent did not require ECMO; however, only 5 of 20 patients who had pulmonary hypoplasia associated with CDH responded positively to HFOV and did not require ECMO. A smaller series of 12 infants, described by Stoddard and colleagues, showed much better outcomes with HFO. Eleven of the 12 babies with CDH did not require ECMO and ultimately survived. Migliazza and colleagues retrospectively reviewed 111 babies with CDH treated with early HFOV for both preoperative stabilization and postoperative care. They saw a 69.4% survival overall, compared to a predicted 69% survival based on the CDH Study Group formula. A 2007 review summarizing “best-evidence practice strategies” discussed HFOV; they found no consistent approach and no new evidence supporting HFOV over conventional approaches to respiratory support.
The sole RCT evaluating the presumed benefits of HFOV in infants with CDH failed to recruit a sufficient number of subjects and was abandoned when an interim analysis showed no hint of benefit of HFOV as applied in that trial. Forty-one patients (45%) randomized to conventional mechanical ventilation died/ had BPD compared with 43 patients (54%) in the high-frequency oscillation group. Patients initially ventilated by conventional mechanical ventilation were ventilated for fewer days (P = 0.03), less often needed extracorporeal membrane oxygenation support (P = 0.007), inhaled nitric oxide (P = 0.045), sildenafil (P = 0.004), had a shorter duration of vasoactive drugs (P = 0.02), and less often failed treatment (P = 0.01) as compared with infants initially ventilated by high-frequency oscillation.
Despite some reports of success, overall, HFV has not been very successful in the treatment of severe pulmonary hypoplasia associated with CDH, at least as an independent treatment. As with other forms of pulmonary hypoplasia, however, HFV often can stabilize critically ill patients until their ultimate prognosis becomes clear. Another common use of HFV is in conjunction with iNO as a treatment for severe respiratory failure secondary to persistent pulmonary hypertension. Detailed discussion regarding the current evidence to support this combined therapy in preterm and term neonates can be found in Chapter 14 .
Clinical Guidelines
Most clinical guidelines are, by their nature, arbitrary; they reflect the experiences, biases, and, at times, idiosyncrasies of their authors. Many clinicians do not consider HFV first-line therapy in the neonatal population; however, many quickly move to it when problems develop during CMV. Some practitioners use HFV early in the course of uncomplicated RDS. Likewise, some wean to extubation from HFV. Others choose a return to CMV prior to extubation. Because of these many clinical variations and the lack of data from which to generalize, the following guidelines must be tempered by experience and modified as new information becomes available. What follows is a description of HFV use in a variety of situations, using two different HFV strategies: (1) limiting pressure exposure, which is used in air leaks, CDH, and most other rescue situations, and (2) optimizing lung volume, which is used in RDS or other conditions in which diffuse atelectasis is a major issue.
Limiting Pressure Exposure
High-Frequency Jet Ventilators
The only high-frequency jet ventilator currently in general use for neonates is the Bunnell Life Pulse.
HFJV settings will depend on the clinical condition. For air leak, the lowest possible rate should be used, which will provide the longest expiratory time. A rate of 240 is often effective in larger infants, 320 to 360 in small preterm infants. Inspiratory time should be left at 0.02 unless a very large infant is being treated, in which case a longer inspiratory time may be needed to generate the required PIP. As is true with both conventional and oscillatory ventilation, mean airway pressure supports end-expiratory lung volume. During HFJV, this is mainly controlled by using PEEP adjustment. Sufficient PEEP is essential for maintenance of lung volume; use of too low a PEEP is a common mistake with HFJV. For air-leak syndromes, a conventional sigh breath should not be used. For other conditions a rate of 420 is usually preferred for preterm infants. A slower rate of 280 to 360 is appropriate for large infants, particularly if there is increased airway resistance, which increases the time needed for passive exhalation. PIP should be sufficient for CO 2 elimination. A transcutaneous monitor to continuously assess CO 2 levels is very important for HFJV and HFOV, as overventilation may occur rapidly, with decreased cerebral blood flow and an increased risk of brain injury and pulmonary air leak.
For nonhomogeneous lung disease with atelectasis and overdistention, a CMV “sigh” rate of 2 to 6 inflations/min may help open atelectatic areas. A common error with HFJV is to use a CV rate instead of appropriate PEEP to increase mean airway pressure. Generally speaking, if addition of a sigh breath improves oxygen saturation, this suggests that end-expiratory lung volume, which is supported by mean airway pressure, is too low. During HFOV with the Dräger BabyLog in an animal model, sigh breaths were useful during lung recruitment but not after recruitment was complete. The PIP of the CMV rate should approximate a pressure that would provide a normal V T of 4 to 6 mL/kg.
Whether or not the sigh breaths interrupt the cycling of the high-frequency jet ventilator is not as important as the V T delivered. Once HFJV settings are established, mean airway pressures should be adjusted as necessary to maintain a balance between the lowest possible pressure and the oxygen exposure.
After HFJV is initiated, some patience is required. During HFJV, airway pressures equilibrate more slowly than during CMV. One must allow adequate time for the system’s servomechanisms to adjust the HFJV driving pressure to achieve the targeted pressures. Patients usually stabilize within 15 to 30 minutes. Continuous CO 2 monitoring is important during this time. After this initial equilibration period, interval arterial blood gases are measured. Usually CO 2 elimination improves, and it may do so at lower mean airway pressures. Oxygen requirements may transiently increase. Because this strategy is designed to minimize pressure exposure, increases in FiO 2 may be necessary to eliminate air leaks. Because the effectiveness of HFJV depends on the ability of the jet of gas to stream down the center of the airway unimpeded, it is important to ensure that the head is in midline and the tip of the endotracheal tube is at least 1 cm above the carina. If the head is turned to the side, the jet stream will hit the wall of the trachea, rendering it less effective and possibly contributing to mucosal injury. If the tube is too close to the carina, the flow of gas may be preferentially directed to one or the other main stem bronchus. Most commonly, HFJV is a rescue therapy; relatively short-term exposure to HFJV (generally a few days) often will result in substantial clinical improvement. As patients improve, HFJV PIP can be decreased in decrements of 1 to 2 cm H 2 O while acceptable pH and PaCO 2 values are maintained. One should also aim to decrease FiO 2 levels as arterial oxygen saturation values allow. Be sure that adequate PEEP is maintained, even in the presence of air leak. Even with a low-pressure strategy, lung end-expiratory volumes must be supported adequately. When HFJV PIP values are below 18 to 20 cm H 2 O and FiO 2 values fall below 0.4, consider extubation to noninvasive ventilation.
If the patient is returned to CMV prior to extubation, set the jet ventilator to standby mode. Set the CMV rate to 60 inflations/min (an arbitrary number) and wean based on patient–ventilator interaction. Adjust CMV PIP levels to deliver V T s of 4 to 6 mL/kg by using a volume-targeted CMV mode. Adjust FiO 2 levels as necessary to maintain arterial oxygen saturation values in the desired range. If, after returning to CMV, the patient’s general condition worsens or FiO 2 or PaCO 2 levels increase significantly, return to HFJV for at least another 24 hours.
High-Frequency Oscillatory Ventilators
In the United States, the most commonly used neonatal high-frequency oscillatory ventilator is the SensorMedics 3100A. With this device, in contrast to HFJV, use of an endotracheal tube adapter is not necessary. Initial HFOV frequency for a premature infant is commonly set between 10 and 15 Hz. Generally, there is seldom a need to change the frequency; however, if the frequency is altered, it is important to be mindful of the effect of frequency on effective V T discussed earlier in this chapter. This ventilator’s “power” control sets the amplitude of its airway pressure oscillations (ÄΔ), the prime determinant of CO 2 removal. Increasing airway pressure amplitude increases chest wall movement and decreases PaCO 2 values, sometimes dramatically. Use of a transcutaneous monitor will provide important data on minute-to-minute gas exchange. Decreasing airway pressure amplitude decreases chest wall movement and increases PaCO 2 values. Initially, the amplitude control is set at a level that adequately produces chest wall vibration or “jiggling” of the chest and abdomen; at times it can be as high as 35 to 40 cm H 2 O when HFOV is used in rescue mode. Some physicians simply use a visual assessment as to adequacy of the chest wall movement to set the initial amplitude value, and others use an amplitude value of approximately double the mean airway pressure as a starting point and adjust as necessary for adequate chest wall movement. After assessment of the initial PaCO 2 value on HFOV, the amplitude is then adjusted up or down as necessary to produce the desired PaCO 2 levels. If a transcutaneous monitor is not available, assessment of PaCO 2 values every 15 minutes will be needed until the patient is stabilized within the goal range. Continued vigilance is essential, because changes in lung compliance may occur quite rapidly and result in large swings in PaCO 2 because of the geometric relationship of V T and CO 2 removal.
Outside the United States, several HFOV devices have the ability to monitor V T and display a calculated value for CO 2 removal, termed DCO 2 . Tracking this number allows the clinician to detect changes in ventilation and respond quickly, similar to what tracking transcutaneous CO 2 allows. Even more attractive is the ability of some of these devices to maintain a target V T by means of a volume guarantee (VG) mode, analogous to conventional VG. With HFOV + VG, once an appropriate V T is identified, that V T can be maintained despite changes in lung mechanics by automatic adjustment of pressure amplitude, analogous to adjustment in PIP with CMV. When the VG mode is used with HFOV, changes in frequency will not produce the usual changes in minute ventilation, because the V T will be unaffected by the frequency change. As of this writing, there is limited information about the effectiveness of this refinement, but it promises to reduce the risk of hypocarbia during HFOV.
During HFOV (as well as HFJV), mean airway pressure is the main determinant of lung volume. Small changes in <SPAN role=presentation tabIndex=0 id=MathJax-Element-6-Frame class=MathJax style="POSITION: relative" data-mathml='P¯aw’>P⎯⎯⎯awˉPaw P¯aw ˉPaw P ¯ aw can produce large changes in lung volume, either overdistention or atelectasis. The airway pressures are measured within the oscillator circuit, not in the endotracheal tube or proximal airway. These pressures may or may not reflect the actual pressures within the patients’ airways. Though mean airway pressure is relatively consistent, oscillation pressures are rapidly damped across the endotracheal tube and further within the airways ( Fig. 22-10 ). <SPAN role=presentation tabIndex=0 id=MathJax-Element-7-Frame class=MathJax style="POSITION: relative" data-mathml='P¯aw’>P⎯⎯⎯awˉPaw P¯aw ˉPaw P ¯ aw should exceed that used during CMV by approximately 2 to 3 cm H 2 O when using a 33% inspiratory time (1:2 I:E ratio). Owing to the flow characteristics of the 1:2 I:E ratio, this will provide a <SPAN role=presentation tabIndex=0 id=MathJax-Element-8-Frame class=MathJax style="POSITION: relative" data-mathml='P¯aw’>P⎯⎯⎯awˉPaw P¯aw ˉPaw P ¯ aw approximately equal to what was given on CMV. This gradient does not exist when a 1:1 ratio is used, as is common with some devices used outside the United States. In theory, the longer expiratory time of the 1:2 I:E ratio means that the expiratory flow and thus pressure during the active exhalation is lower than during the inspiratory phase, making airway collapse less likely to occur. Mean airway pressure can then be adjusted depending on chest radiographs and oxygenation. Once the patient stabilizes or starts to improve, airway pressures should be decreased, as long as FiO 2 is below 0.30 and PaCO 2 values are normal. The ÄΔ should be lowered if PaCO 2 levels are below target. During HFOV weaning, if FiO 2 increases, consider a 1-to 2-cm H 2 O increase in <SPAN role=presentation tabIndex=0 id=MathJax-Element-9-Frame class=MathJax style="POSITION: relative" data-mathml='P¯aw’>P⎯⎯⎯awˉPaw P¯aw ˉPaw P ¯ aw to stabilize lung volume. Some advocate intermittent lung volume recruitment maneuvers when FiO 2 increases to reestablish adequate end-expiratory lung volume. If things do not improve or worsen, a chest radiograph may be needed. When the FiO 2 is below 0.3 to 0.4, the chest radiograph is significantly improved, and the <SPAN role=presentation tabIndex=0 id=MathJax-Element-10-Frame class=MathJax style="POSITION: relative" data-mathml='P¯aw’>P⎯⎯⎯awˉPaw P¯aw ˉPaw P ¯ aw is 7 to 9 cm H 2 O, infants can be extubated to noninvasive ventilation. In some situations or for heavily sedated infants, return to CMV may be needed.
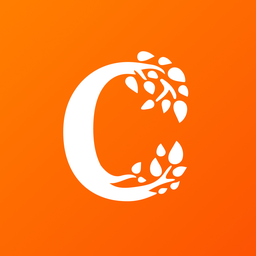
Full access? Get Clinical Tree
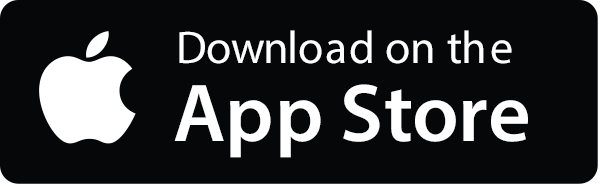
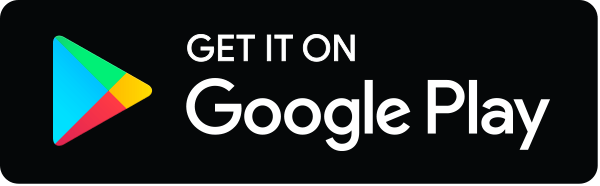