Key Points
- •
Hematopoietic stem cell transplantation (HSCT) can correct many primary immune deficiencies (PIDs) by providing a normal source that produces functional leukocytes to replace the defective cells.
- •
Best outcomes are obtained when using matched sibling donor bone marrow, although results using matched unrelated adult or umbilical cord blood donors or haploidentical (parental) donors continue to improve.
- •
Multiple factors affect outcomes, include the specific PID being treated, the general health of the recipient, presence or absence of intercurrent infections, the choice of pretransplant conditioning regimen used to facilitate engraftment and the source of donor hematopoietic stem cells.
- •
Several clinical trials of gene therapy – autologous transplantation of genetically corrected hematopoietic stem cells – using murine retroviral vectors showed significant immune reconstitution. However, there was a high incidence of vector-related leukoproliferative complications.
- •
Current trials mostly use lentiviral vectors, which may be more effective and safer than the murine retroviral vectors, further improving outcomes for gene therapy for PID.
Several forms of primary immune deficiency (PID) result from mutations of genes involved in the production, function or survival of leukocytes. Therefore, these PIDs can be treated by allogeneic hematopoietic stem cell transplantation (HSCT) that provides patients with a new source of genetically normal leukocytes. The first successful clinical allogeneic bone marrow transplant (BMT) was performed in 1968 for a patient with severe combined immune deficiency (SCID), resulting in sustained reconstitution of immunity that has lasted now for several decades. Since that time, allogeneic HSCT has become the standard of care for patients with SCID and is often used for patients with other severe, life-threatening forms of PID. Patients with less severe PIDs, especially those primarily affecting production of antibodies that can be replaced with intravenous gammaglobulin administration, generally are not treated with HSCT.
The major barriers to allogeneic HSCT are immunologic. In solid organ transplantation, a recipient’s T cells can reject the organ donor’s cells. Graft rejection is less of a problem in severely immune deficient recipients, such as patients with SCID who lack T lymphocytes, and in some cases also B and NK cells. However, in some of the PIDs that retain partial function of the immune system, a significant risk for graft rejection remains. In most cases, transplant recipients need partial or essentially complete ablation of their endogenous immune and hematopoietic systems to achieve enduring engraftment of donor HSCs. Definition of optimal pretransplant conditioning regimens remains one of the major areas of discovery in the field.
The flipside of graft rejection, which is mostly limited to HSCT and only rarely encountered in solid organ transplant, is graft versus host disease (GvHD), where alloreactive T cells from the donor that are given with the allogeneic bone marrow can, in effect, reject the recipient’s cells. GvHD can be severe, chronic and even fatal; complications increase in frequency and severity with increasing degree of mismatch between the donor and the recipient. Efforts to prevent or suppress GvHD, by administration of immune suppressive medications or by depleting the donor’s mature T lymphocytes from the graft, often result in prolonged immune deficiency that poses high risks for opportunistic infections, especially beyond the infant period when patients may bear latent herpesviruses, BK virus or adenovirus.
Severe Combined Immune Deficiency
Severe combined immune deficiency is the most severe of the PIDs, with inherited absence of T and B cell function (and variable NK activity). In the absence of medical intervention, infants with SCID suffer a high rate of early mortality from infections. SCID is a clinical phenotype which may result from defects in any one of more than 12 genes ( Box 16-1 ). The clinical research associated with the treatment of human SCID has produced many of the major advances in the field of HSCT ( Box 16-2 ).
Signaling Defects
Common γ cytokine receptor chain ( IL2RG gene ) (XSCID) (~30%)
JAK3
IL-7Rα
CD3 chains (CD3δ, CD3ε, CD3ζ)
CD45
Recombinase Defects
RAG1 / RAG2
Artemis ( DLCRE1C )
DNA-dependent protein kinase catalytic subunit (DNA-PKcs)
Cernunnos
Metabolic Defects
Adenosine deaminase ( ADA ) (10–15%)
Purine nucleotide phosphorylase ( PNP )
Adenylate kinase 2 ( AK2 )
First successful human BMT (1968)
First use of matched unrelated donor (MUD) bone marrow (1973)
First transplant with haploidentical (parent) bone marrow (1975)
First approach using gene therapy (1990)
First ‘cure’ using gene therapy (2000)
Allogeneic HSCT can be curative for SCID when bone marrow from a human leukocyte antigen (HLA)-matched sibling donor (MSD) is used, with most centers reporting greater than 90% long-term survival. PID patients who receive an allogeneic HSC transplant from an HLA-MSD generally achieve high rates of immune reconstitution and good quality of life. For patients with SCID, HLA-MSD marrow can be infused without chemotherapy or radiation ‘conditioning’, and will appropriately restore T cell function in most patients. Most other PIDs require full or partial marrow ‘conditioning’ for proper engraftment of donor cells without rejection.
By contrast, HSCT from HLA-mismatched donors (e.g. a haploidentical/haplodisparate parent) carries a significant risk of severe GvHD, because the donor’s T cells contained in the graft would react against the non-shared HLA proteins (such as those inherited from the other parent). To prevent this, methods to deplete T lymphocytes from bone marrow in order to limit GvHD risks were developed in the mid 1970s ( Box 16-3 ). Using such approaches to deplete T cells, marrow from parental donors, who are only matched by one HLA haplotype and therefore mismatched for a full HLA haplotype, has been frequently used for SCID patients lacking an HLA-matched donor; 50% to 80% of SCID patients have realized long-term survival. The lower success rate with haploidentical transplants may reflect the morbidity and mortality associated with: (1) the increased risks of graft rejection and/or GvHD; (2) the T cell depletion that is utilized to prevent GvHD which slows immune reconstitution and prolongs the period of increased susceptibility to infections; and (3) the adverse effects secondary to the possible use of chemotherapy to make space and eliminate residual immunity.
Complement-mediated lysis
Counterflow centrifugation elutriation
Density gradients
Polyclonal antibodies (ALG, ATG)
Monoclonal antibodies (anti-CD2, anti-CD3, anti-CD4, anti-CD5, anti-CD6, anti-CD7, anti-CD8, anti-CD52, TcRαβ, CD45RA)
Soy bean agglutinin/E-rosette with sheep red blood cells
Stem cell enrichment (e.g. anti-CD34)
One of the continuing controversies about the optimal approach to haploidentical HSCT for SCID concerns the necessity, or lack thereof, of pretransplant conditioning with chemotherapy or other immune suppressive medications to facilitate long-term engraftment of donor HSCs. In the absence of conclusive data from randomized clinical trials, there are strong advocates of either of these approaches.
A few very active centers have performed haploidentical transplants for SCID patients for more than three decades, successfully giving T cell-depleted bone marrow from a parent without any preconditioning therapy or immune suppressive medicines to prevent GvHD. Most of these patients have had successful T cell immune reconstitution lasting decades, although it will take longer observation to assess the durability of T cell function. However, more than half of them have had inadequate B cell reconstitution and were reported still to require regular administration of intravenous gammaglobulin, possibly indicating a failure to engraft sufficient numbers of HSCs in the absence of marrow conditioning.
Use of cytoreductive conditioning prior to HSCT for SCID typically leads to donor cell chimerism in all lineages, indicating replacement of the endogenous HSCs with those of the donor. This full donor engraftment may lead to more sustained production of new T cells and may lead to increased numbers and activity of B and NK cells. However, the acute and long-term toxicities of the chemoablative regimens may lead to increased toxicity and mortality in the initial period, especially in patients with preexisting infections, and may cause long-term growth, endocrinologic and neurocognitive abnormalities.
Historically, less has been done using matched unrelated donor marrow (MUD) or cord blood for transplantation of SCID patients who do not have an HLA-MSD. HSC transplantation using MUD or cord blood carries increased risks for GvHD, compared to the use of marrow from an MSD. The frequency of severe GvHD in MUD marrow and cord blood HSC transplantation is in the range of 25–50% and 20–40%, respectively. In addition, HSC transplantation with cord blood has a risk for engraftment failure between 10% and 20%. These increased complications are likely to be due to more mismatches for non-HLA (minor) antigens. Generally, full cytoablation and GvHD prophylaxis (i.e. post-transplant immune suppression) are needed to prevent graft rejection or GvHD.
In the past few years, a consortium of investigators from North America involved in the clinical care of patients with severe PIDs has organized as the Primary Immune Deficiency Treatment Consortium (PIDTC) to compile data on treatment and outcomes, mainly focussed on hematopoietic transplants. Results were analyzed retrospectively for transplants performed between 2000 and 2009 in 240 patients with typical or ‘classical’ SCID. Outcomes for infants treated at less than 3.5 months of age were excellent (94% 5-year survival), independently of the source of HSC used. For patients older than 3.5 months of age at the time of transplant, the presence of ongoing infections, mostly viral, led to poorer survival (50% 5-year survival). Among patients who were infected at the time of HSCT, the outcome was worse in those who received pretransplant conditioning (31.2% 5-year survival) than in those transplanted without conditioning (61.4% 5-year survival). Nevertheless, for surviving infants, T and B cell functions were more frequently better in those who received pretransplant conditioning, which raises a conundrum in defining the best approach ( Box 16-4 ). Importantly, newborn screening for SCID is now available in many States and may permit rapid identification of affected babies, who can be referred to HSCT before developing infections. While this is expected to result in improved outcome, the role (and intensity) of pretransplant conditioning in this group of very young infants remains to be defined.
MSD when available for all, without conditioning
Infants 3.5 months or less: MSD, MMFD, MUD (adult or cord blood) or autologous/gene therapy; with or without conditioning, per center experience
Infants > 3.5 months without resistant viral infection: MSD, MMFD, MUD (adult or cord blood), or autologous/gene therapy; with or without conditioning, per center experience
Infants > 3.5 months with resistant infection: MSD, MMFD, MUD (adult or cord blood) or autologous/gene therapy; without conditioning. May develop full or partial T cell immunity, but good B and NK cell function unlikely. Second, conditioned transplant may be considered at time of improved health to improve immunity
MSD, Matched sibling donor; MMFD, Mismatched family donor, typically parent; MUD, Matched unrelated donor.
Because SCID is rare, and may be caused by various genetic defects, few studies have analyzed the outcome of HSCT in specific forms of SCID. An international consortium has reported on the outcome of HSCT in 106 patients with ADA deficiency. Overall survival after HSCT from MSD or matched family donors (86% and 81%, respectively) was better than from MUDs (66%; P < .05) or haploidentical donors (43%; P < .001). Survival was superior for patients who received unconditioned transplants than for those who received myeloablative conditioning (81% vs 54%, respectively; P < .003). However, unconditioned transplantation was associated with a higher risk of non-engraftment. Long-term studies showed that most surviving patients attained durable immune reconstitution.
Defects of V(D)J recombination due to RAG, Artemis, DNA PKcs, or Cernunnos mutations cause T − B − NK + SCID. In addition, Artemis and DNA PKcs deficiency cause increased cellular radiosensitivity, which may lead to additional toxicity when conditioning regimens are used for HSCT. Schuetz et al have reported on the outcome of HSCT in patients with Artemis mutations vs patients with RAG1/2 deficiency. Survival after MSD HSCT was comparable in the two groups. However, patients with Artemis deficiency had an increased rate of late complications, including poor growth and requirement for nutritional support, autoimmune manifestations, growth hormone deficiency, hypothyroidism and dental anomalies.
Wiskott-Aldrich Syndrome
The pathophysiology, presentation, diagnosis and general management of Wiskott-Aldrich syndrome (WAS) are discussed in Chapter 9 .
Bach and colleagues performed the first sibling HSCT for WAS. MSD HSCT provides the best outcome for WAS patients and is established as the standard of care when available. In recent years, advances in transplant methods have significantly improved outcome of MUD HSCT also in WAS patients less than 5 years old. In particular, in a recent multicenter international study of 194 patients with WAS who received HSCT in the period 1990–2009, 5-year survival was 84%, and it was 73.3% in those who received MUD HSCT at less than 5 years of age.
With current approaches, the majority (72%) of WAS patients attain full chimerism after HSCT. However, mixed chimerism may be associated with complications. In particular, post-HSCT autoimmune disease is frequently observed. Host WASp-deficient B cells that persist after transplantation due to mixed donor chimerism may not respond fully to regulatory cues and produce autoantibodies. Furthermore, low myeloid chimerism is associated with an increased risk of persistent thrombocytopenia. Overall, HLA-MSD HSCT in patients who are less than 5 years of age and achieve full donor chimerism has the best outcome, but if an MSD is unavailable, a matched unrelated donor HSCT is a viable option.
Chronic Granulomatous Disease
The pathophysiology, presentation, diagnosis and general management of chronic granulomatous disease (CGD) are discussed in Chapter 11 (and reference ). It is estimated that the median lifespan of patients with CGD is 20 to 25 years and the mortality rate is 2% to 5% per year. Since many CGD patients live into adulthood, in contrast to the near certain early mortality of SCID, decisions are more difficult concerning the potential benefits of transplant versus the risks that are associated with a myeloablative HSCT. Theoretically, reduced intensity conditioning decreases the toxicity associated with the conditioning regimen and T cell depletion decreases the risks for GvHD. Recently, a multicenter European study using reduced intensity conditioning and matched sibling or matched unrelated donors for CGD reported excellent outcomes for 56 CGD patients; overall survival was reported as 93% with event-free survival (surviving with donor engraftment) at 89%.
Hemophagocytic Lymphohistiocytosis
Hemophagocytic lymphohistiocytosis (HLH) is a rare disorder that is characterized by highly activated macrophages and lymphocytes. Primary or familial HLH is inherited as an autosomal recessive disease, while secondary HLH is an acquired form that is usually associated with a viral illness. While initially considered to be a malignant disorder of histiocytes, more recent understanding of HLH classifies it as a PID, with defects in genes involved in transport and/or release of cytolytic granules, such as perforin, MUNC 13-4 , STX11 , STXBP2 . Inability to kill activated cells results in persistent stimulation of the immune system with increased production of IFN-γ and uncontrolled activation of macrophages.
The first curative HSCT for HLH was performed in 1986. Currently, standard treatment for the familial type involves induction immunochemotherapy with etoposide, dexamethasone and cyclosporin A, followed by HSCT. An earlier clinical trial, HLH-94, showed that the outcomes with MUD HSCT were equivalent to those with HLA-MSD HSCT (67% vs 68%). Patients who received transplants from mismatched related donors (usually haploidentical) had a survival rate of 43%. More recently, significant improvement of outcome has been reported after HSCT for HLH with use of a reduced intensity conditioning (RIC) regimen. In a series of 40 patients who received HSCT for HLH at Cincinnati Children’s Hospital, 3-year overall survival was 43% for those who received fully myeloablative conditioning (MAC) vs 92% for those who received RIC. This difference was mostly due to increased risk of early death (< 6 months after HSCT) in patients receiving MAC. Although RIC is associated with an increased rate of mixed chimerism, levels of 10–20% donor chimerism apparently suffice to control HLH.
Other Primary Immune Deficiencies Amenable to Hematopoietic Stem Cell Transplantation
HSCT is the treatment of choice also for severe defects that affect late stages of T cell development (such as ZAP-70 deficiency and MHC class II deficiency), other forms of combined immunodeficiency (X-linked hyper-IgM syndrome, DOCK8 deficiency), immunodeficiency with severe immune dysregulation (IPEX), and X-linked lymphoproliferative disease (XLP). At variance with SCID, these PIDs are characterized by the presence of autologous T cells, requiring use of conditioning in preparation for HSCT. Less severe PIDs, such as X-linked agammaglobulinemia (XLA), common variable immune deficiency, selective antibody defects, etc., have not been routinely subjected to HSCT thus far, since the risks of HSCT may exceed those of the underlying disorder.
Gene Therapy Using Hematopoietic Stem Cells
Gene Therapy for ADA-Deficient SCID
Gene therapy was conceived as an alternative to allogeneic HSCT in which a patient’s own HSCs would have a normal copy of the disease-related gene inserted ( Figure 16-1 ). Autologous gene therapy should avoid the immunologic complications of allogeneic HSCT (graft rejection and GvHD) but could yield the same clinical benefits. Based on the known therapeutic effects from non-myeloablated MSD transplants for SCID with only low engraftment of donor HSCs, it was postulated that in SCID there would be selective lymphoid expansion from a small number of gene-corrected HSCs that could amplify the effects of low efficiency gene transfer.

Six clinical trials of gene therapy for ADA-deficient SCID were performed in the 1990s. All of these studies used retroviral vectors to transfer a normal ADA cDNA, targeting either mature T lymphocytes that developed after PEG-ADA therapy, or CD34 + HSCs from autologous bone marrow or umbilical cord blood. While there were no serious adverse events from the gene transfer in any of the subjects, there were also no significant clinical benefits. Overall, survival and immune function in these subjects were comparable to those in patients receiving only PEG-ADA therapy.
During the 1990s, incremental progress was made with techniques of gene transfer and stem cell culture. Additionally, the use of RIC prior to allogeneic HSCT was shown to allow a moderate degree of engraftment of donor cells, with significantly reduced acute toxicity. Such non-myeloablative conditioning was postulated to be beneficial in the autologous gene therapy setting ( Figure 16-2 ). Based on these advances, second-generation clinical trials of gene therapy for SCID were begun in the late 1990s.

The investigators at the San Raffaele Telethon Institute for Gene Therapy in Milan, Italy, made a major advance in the field with their clinical trial of gene therapy for ADA-deficient SCID. Initial results were reported in 2002 on two ADA-SCID infants treated using retroviral-mediated ADA gene transfer to bone marrow CD34 + cells. Two important variables were changed, compared to earlier trials. First, the patients were given RIC, using a moderate dosage of busulfan (4 mg/kg, approximately of ‘full dose’) to eliminate some of the endogenous HSCs prior to infusion of the gene-corrected bone marrow cells. Second, the patients were not given PEG-ADA enzyme replacement therapy, which was expected to allow the maximum selective advantage of gene-corrected T cells to manifest. Indeed, over the first 6 to 9 months, immune function was largely restored, with the development of antigen-specific T cell responses and antibody production. Measurements of gene marking showed that 75–100% of T, B and NK cells contained the transferred gene, consistent with the strong purported selective advantage for ADA-expressing lymphocytes. At the same time, 1–10% of myeloid cells contained the gene, which was a level significantly higher than that seen in earlier studies in which cytoreductive chemotherapy was not given.
Further results of this approach have now been reported from Milan, with 8 of 10 subjects realizing excellent and sustained immune reconstitution. Similar studies at University College London/Great Ormond Street Hospital in London, UK, and in the USA at Children’s Hospital Los Angeles, the University of California, Los Angeles, and the National Human Genome Research Institute, NIH, have led to similar clinically beneficial results. Of at least 40 ADA-SCID patients treated by gene therapy using retroviral vectors and non-myeloablative conditioning in these studies, all are surviving and approximately 75% have sufficient immune restoration to remain well without other treatment, such as enzyme replacement therapy or allogeneic HSCT. No complications from genotoxic effects of the vectors have been observed in any, in contrast to findings in other diseases (see below). Thus, for ADA-deficient SCID patients lacking an HLA-MSD, gene therapy has become a proven therapeutic modality.
Gene Therapy for XSCID
The next PID approached as a candidate for gene therapy was X-linked SCID (XSCID), caused by mutations of the common cytokine receptor γ chain (γc or IL2Rγ). Investigators at the Hopital Necker- Enfants Malades in Paris, France, instituted a clinical trial of retroviral-mediated transfer of a normal human γc cDNA into CD34 + cells from bone marrow of infants with XSCID. In this trial, the transduced CD34 + cells were reinfused without any prior cytoreduction, counting on the very potent selective survival advantage of the γc-corrected lymphoid cells to repopulate the immune system from a low level of gene-modified HSCs. No adverse events were noted in the initial years in 9 treated subjects. The γc gene was present and expressed in T, NK and B cells. Over the initial 2 to 5 months, 9 of 10 infants developed normal numbers of T and NK cells, with good immune function. While B cell numbers remained low, protective levels of antibodies were produced and some of the subjects were removed from routine gammaglobulin treatment. The subjects were in good health over the first 1 to 2 years, without opportunistic infections, and were growing and developing without protective isolation. Ten XSCID infants were treated in a similar clinical trial by investigators at the Institute of Child Health, University College London, UK, with similarly good outcome.
Longer-term follow-up of subjects in both the French and British trials was published and reported sustained T cell immunity in those achieving initial reconstitution. However, B cell function allowing independence from IVIG replacement therapy was achieved in only 4 of 7 and 5 of 10, respectively, with essentially no gene-corrected B cells detected and a paucity of memory B cells; apparently, gene-corrected T cells are not of adequate help to induce full maturation and function in the non-gene-corrected B cells.
However, 5 subjects from these two trials subsequently developed a leukemia-like complication, with escalating white blood cell counts, 2.5–5 years after the gene therapy procedures. Four of these subjects have been successfully treated for the lymphoproliferative syndrome and have retained the benefits of the gene therapy on immune reconstitution, but one died as a result of this complication. Investigations have implicated the process of insertional oncogenesis, in which the retroviral vector integrates semirandomly into different chromosomal sites in each transduced cell; integrants adjacent to cellular genes that mediate proliferation or survival may be inappropriately trans -activated by transcriptional elements of the vector, such as the potent enhancers present in the long terminal repeats (LTRs). It remains uncertain why the complication of insertional oncogenesis with lymphoproliferation was seen in 25% of the XSCID subjects but in none of the more than 40 subjects with ADA-deficient SCID.
Faced with these mixed results with life-saving immune reconstitution in the majority of XSCID subjects using gene therapy, but the potential for a severe treatment-related complication, the relative risks and benefits need to be compared to the current therapeutic alternative for these subjects – allogeneic HSCT from a haploidentical or MUD donor. SCID patients with allogeneic donors other than HLA-matched siblings have had survival rates of 50% to 80% with restored T cell immunity, as discussed previously. However, more than half of these patients may fail to produce protective antibodies, and there are risks of GvHD and post-transplant Epstein-Barr virus (EBV)-driven lymphoproliferative disease (LPD). Gene therapy for XSCID using retroviral vectors led to immune restoration in 90% 18 of 20) of patients, but with a 25% incidence of LPD.
Notably, this same approach for gene therapy has been applied to older XSCID subjects, most of whom had undergone prior HSCT from haploidentical donors that resulted in only partial immune reconstitution with ongoing poor health. These adolescent subjects showed minimal responses to the gene therapy, suggesting that their older age was associated with inadequate thymic function to support de novo lymphopoiesis; it is uncertain if the prior transplants were associated with GvHD, which is known to impair thymic function.
The way forward for gene therapy for XSCID lies in evaluating new vector designs that lack the strong LTR enhancer elements (so-called ‘self-inactivating’, or ‘SIN’ vectors), which have been shown by in vitro and murine transplant studies to have significantly lowered risks for causing insertional oncogenesis. Ideally, these improved vectors will lead to similar levels of immune reconstitution, with minimal or no occurrences of insertional oncogenesis. One such trial with use of a SIN γ-retroviral vector to treat infants with XSCID without conditioning regimen is currently underway at five collaborating institutions in London, Paris, Boston, Cincinnati and Los Angeles. Initial results have shown ability to attain T cell immune reconstitution, and no clonal proliferations have been observed so far, although follow-up is still limited. More recently, De Ravin and colleagues opened a second clinical trial to treat older, partially reconstituted XSCID patients, in the present form using a lentiviral vector and RIC (6 mg/kg busulfan). Initial results indicate some improvements of B cell immunity and overall wellbeing.
Gene Therapy for CGD
Another major PID that has been approached in several gene therapy clinical trials is CGD. The cDNA for the oxidases responsible for the X-linked form (gp91 phox ) and most common autosomal forms (p47 phox , p67 phox or p22 phox ) were cloned and placed into retroviral vectors. Preclinical studies using patient-derived cells and murine gene knockout models provided evidence that gene transfer could at least partially restore the defective oxidase function in myeloid cells. Initial trials in X-linked CGD (the most common form of the disease) used murine leukemia virus (MLV)-based retroviral vectors, targeted G-CSF mobilized CD34 + peripheral blood stem cells (PBSC) and did not administer cytoreductive chemotherapy. Neutrophils were produced in vivo in multiple subjects that had their functional activity restored, based upon sensitive flow cytometric assays, but these were present at very low frequencies and only transiently.
A later study performed at the German Cancer Research Center added cytoreductive conditioning with 8 mg/kg of busulfan (approximately two times higher than the dose used in the ADA-deficient SCID studies) and used a retroviral vector derived from the murine spleen focus forming virus (SFFV) which has LTRs that are highly potent in myeloid progenitor cells expressing the normal human gp91 phox . Two young men in their twenties with X-CGD and lifelong histories of chronic infections poorly responsive to intensive medical therapy were treated. They had clinically beneficial responses to the treatment with resolution of the longstanding infections. The production of gene-corrected, oxidase (+) neutrophils was readily detected; the frequencies of gene-containing neutrophils increased from 20% in the first few months after transplant to levels exceeding 60% over the next few months. Both patients developed monosomy 7 and myelodysplasia. One of these patients died as a result of gastrointestinal infection, at a time when there was loss of expression of oxidase activity in neutrophils, despite the continued presence of gene-containing cells.
The highly dichotomous outcome of clinical benefit followed by severe adverse event in these CGD patients is highly reminiscent of the XSCID studies. For CGD, the use of a vector with a potent myeloid-type LTR promoter led to myeloproliferation, while there was lymphoproliferation in the XSCID studies where the MLV LTR is more active in lymphoid cells. It is known from research on wild-type retroviruses that the virus’s LTRs may play a major role in defining the disease tropism. Retroviral vectors used to drive high-level expression of a transgene in a specific lineage may predispose that lineage to insertional oncogenic effects.
The unexpected complications that occurred in these patients treated for PID led to major increases in the understanding of these risks, their underlying mechanisms and potential ways to overcome them. Some of these improved approaches will be discussed below. A lentiviral vector has been developed to express the gp91 phox cDNA under transcriptional control of a chimeric promoter with expression relatively specific to mature myeloid cells; this vector could be active in granulocytes and monocytic cells but have a reduced potential to transform stem and progenitor cells. A multinational clinical trial using this new vector has recently started in Europe, with a US trial planned.
Gene Therapy for WAS
A clinical trial for WAS performed in Germany using a first-generation retroviral vector led to sustained engraftment and correction of WASp expression in lymphoid and myeloid cells and platelets in 9 out of 10 patients treated, resulting in improved immunologic and hematologic status. However, 7 of these 9 patients developed leukemia due to insertional mutagenesis, which makes this approach unacceptable. Initial results from a trial for WAS using a lentiviral vector with the endogenous WAS gene promoter at San Raffaele Telethon Institute for Gene Therapy in Milan, Italy, demonstrated good immune reconstitution, significant improvement in platelet numbers (although they remained subnormal) and no safety problems in the initial period of observation. Another multicenter trial using the same vector but a slightly different conditioning regimen is under way in London, Paris and Boston.
Perspectives for Gene Therapy in Other Primary Immune Deficiencies
Other PIDs that are under extensive study and are subject, or will be subject, to gene therapy clinical trials include SCID due to RAG1, RAG2 or Artemis deficiency, LAD and HLH. Other PIDs that involve defects in cytokine and signaling pathway components, such as the JAK3 kinase and ZAP-70 kinase, CD40 ligand deficiency (X-linked hyper-IgM syndrome), IPEX, IL-7 receptor and XLA will require new approaches to gene therapy to achieve more sophisticated control of the expression of the responsible gene than occurs using constitutive promoters such as viral LTRs.
New Approaches to Gene Therapy Using Hematopoietic Stem Cells
Gene therapy for PIDs has progressed from an essentially ineffective method in the 1990s to its present status, where clear-cut efficacy has been achieved for several disorders, albeit with a risk for a significant degree of side-effects. Further advances will be based upon new insights into HSC biology and new methods for gene transfer. New understandings may eventually support efforts for ex vivo expansion of HSCs, which would allow the selective use of HSCs with favorable vector integration sites.
New major alternatives to the murine γ-retroviral vectors are vectors derived from lentiviruses or foamy viruses. These latter types of retrovirus are of primate origin (HIV-1 lentiviruses from humans and the simian foamy virus from nonhuman primates) and are more efficient for transferring genes into human cells. These vectors can be made lacking the strong LTR enhancers that are problematic in the γ-retroviral vectors. Lentiviral vectors have entered clinical trials for T cells and CD34 + HSCs and are likely to be applied to several PIDs and other genetic blood disorders in the near future. A study in a canine model of LAD showed excellent clinical response using a foamy viral vector to transfer the relevant CD18 cDNA and may also be moving to the clinic. Another important tactic being explored for added safety is the addition to vectors of ‘insulator’ sequences, which are DNA sequences that act as boundaries to prevent transcriptional cross-talk between adjacent genes in the chromosomes.
Gene Correction
All of the gene therapy efforts discussed so far have involved the use of methods for adding a functional copy of a gene to cells (in a nonphysiologic chromosome location). A promising new approach to gene therapy under investigation seeks to correct the disease-causing mutation in a patient’s own gene. Effective gene correction may have key advantages over gene addition. The corrected therapeutic gene would be in its normal location in the chromosomes, and so should be expressed in the normal developmental and quantitative pattern; this may be essential for safe and effective therapy of diseases due to defects in signaling proteins, including XLA, X-linked hyper-IgM syndrome and ZAP-70 kinase deficiency, as discussed above. Gene correction should avoid the problems of insertional oncogenesis from gene addition due to random insertion throughout the genome, resulting in activation of nearby genes.
Cells have multiple ways to repair damage to their chromosomal DNA, including homologous recombination (HR) that can lead to the genetic information on one strand being copied into a homologous, but nonidentical sequence ( Figure 16-3 ). Flooding cells with high concentrations of nucleic acid sequences complementary to an endogenous gene sequence can cause the HR pathways to introduce corrective sequences into a cellular gene, for example to correct the single base-pair change in the human β-globin gene responsible for sickle cell disease. However, early efforts to direct gene repair by HR were limited by very low efficiency. More recently, the efficiency of HR has been markedly improved by the use of transiently expressed site-specific endonucleases targeted to introduce double-stranded DNA breaks near the intended site of HR. A succession of such engineered nucleases (zinc-finger nucleases [ZFNs], homing endonucleases, TALENS and CRISPR/Cas9) can be designed to have DNA recognition domains that bind to unique sites in the genome and introduce a double-stranded break to induce HR, guided by a ‘donor’ oligonucleotide. Gene correction by HR may be developed to achieve correction of the genes involved in PIDs. It will need to be determined whether the efficacy and safety of gene correction provide a better therapeutic window than gene addition methods.
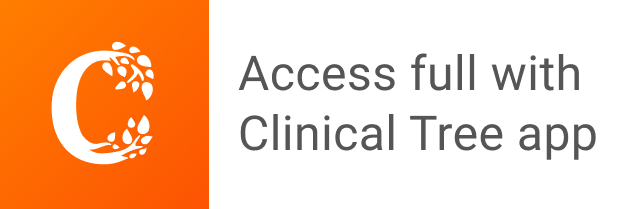