Figure 7-1 Terminology used to describe the pregnancy duration.
Clinicians customarily calculate gestational age as menstrual age. Approximately 280 days, or 40 weeks, elapse on average between the first day of the last menstrual period and the birth. This corresponds to 9 and 1/3 calendar months. A quick estimate of a pregnancy due date based on menstrual data can be made as follows: add 7 days to the first day of the last period and subtract 3 months. For example, if the first day of the last menses was July 5, the due date is 07–05 minus 3 (months) plus 7 (days) = 04–12, or April 12 of the following year. This calculation has been termed Naegele rule. Many women undergo first- or early second-trimester sonographic examination to confirm gestational age. In these cases, the sonographic estimate is usually a few days later than that determined by the last period. To rectify this inconsistency—and to reduce the number of pregnancies diagnosed as postterm—some have suggested assuming that the average pregnancy is actually 283 days long and that 10 days be added to the last menses instead of 7 (Olsen, 1998). The period of gestation can also be divided into three units, each 13 to 14 weeks long. These three trimesters are important obstetrical milestones.
EMBRYO-FETAL GROWTH AND DEVELOPMENT
The complexity of embryo-fetal development is almost beyond comprehension. Shown in Figure 7-2 is a schematic sequence of various organ systems as they develop. New information regarding organ development continues to accrue using modern technologies. For example, imaging techniques help evaluate the role of gene regulation and tissue interaction on eventual 3-dimensional organ morphology (Mohun, 2011). And, Williams and colleagues (2009) described the sequence of gene activation that underlies cardiac development.
Figure 7-2 Embryo-fetal development according to gestational age determined by the first day of the last menses. Times are approximate.
Zygote and Blastocyst Development
During the first 2 weeks after ovulation and then fertilization, the zygote develops to the blastocyst stage, which undergoes implantation 6 or 7 days following fertilization. The 58-cell blastocyst differentiates into five embryo-producing cells—the inner cell mass—and the remaining 53 cells form the placental trophoblast. Details of implantation and early development of the blastocyst and placenta are described in Chapter 5 (p. 88).
Embryonic Period
The conceptus is termed an embryo at the beginning of the third week after ovulation and fertilization. Primitive chorionic villi form, and this coincides with the expected day of menses. The embryonic period lasts 8 weeks, during which organogenesis takes place. The embryonic disc is well defined, and most pregnancy tests that measure human chorionic gonadotropin (hCG) become positive by this time. As shown in Figures 7-3 and 7-4, the body stalk is now differentiated, and the chorionic sac is approximately 1 cm in diameter. There are villous cores in which angioblastic chorionic mesoderm can be distinguished and a true intervillous space that contains maternal blood. During the third week, fetal blood vessels in the chorionic villi appear. In the fourth week, a cardiovascular system has formed, and thereby, a true circulation is established both within the embryo and between the embryo and the chorionic villi. By the end of the fourth week, the chorionic sac is 2 to 3 cm in diameter, and the embryo is 4 to 5 mm in length (Figs. 7-5 and 7-6). Partitioning of the primitive heart begins in the middle of the fourth week. Arm and leg buds are present, and the amnion is beginning to unsheathe the body stalk, which thereafter becomes the umbilical cord.
FIGURE 7-3 Early human embryos. Ovulation ages: A. 19 days (presomite). B. 21 days (7 somites). C. 22 days (17 somites). (After drawings and models in the Carnegie Institute.)
FIGURE 7-4 Drawing of an 18-day Mateer-Streeter embryo shows the amnionic cavity and its relations to chorion and yolk sac. (Redrawn from Streeter, 1920.)
FIGURE 7-5 Three- to four-week-old embryos. A, B. Dorsal views of embryos during 22 to 23 days of development showing 8 and 12 somites, respectively. C–E. Lateral views of embryos during 24 to 28 days, showing 16, 27, and 33 somites, respectively. (Redrawn from Moore, 1988.)
Figure 7-6 Embryo photographs. A. Dorsal view of an embryo at 24 to 26 days and corresponding to Figure 7-5C. B. Lateral view of an embryo at 28 days and corresponding to Figure 7-5E. C. Lateral view of embryo-fetus at 56 days, which marks the end of the embryonic period and the beginning of the fetal period. The liver is within the fine, white circle. (From Werth, 2002, with permission.)
At the end of the sixth week, the embryo is 22 to 24 mm long, and the head is large compared with the trunk. The earliest synapses in the spinal cord develop at 6 to 7 weeks (Kadic, 2012). The heart is completely formed. Fingers and toes are present, and the arms bend at the elbows. The upper lip is complete, and the external ears form definitive elevations on either side of the head. Three-dimensional images and videos of human embryos from the MultiDimensional Human Embryo project are found at: http://embryo.soad.umich.edu/.
Fetal Period
Transition from the embryonic period to the fetal period is arbitrarily designated by most embryologists to begin 8 weeks after fertilization—or 10 weeks after onset of last menses. At this time, the embryo-fetus is nearly 4 cm long (see Fig. 7-6C).
Development during the fetal period consists of growth and maturation of structures that were formed during the embryonic period. Crown-to-rump measurements, which correspond to the sitting height, are most accurate for dating (Table 7-1).
TABLE 7-1. Criteria for Estimating Age During the Fetal Period
12 Gestational Weeks
The uterus usually is just palpable above the symphysis pubis, and the fetal crown-rump length is 6 to 7 cm. Centers of ossification have appeared in most fetal bones, and the fingers and toes have become differentiated. Skin and nails have developed, and scattered rudiments of hair appear. The external genitalia are beginning to show definitive signs of male or female gender. The fetus begins to make spontaneous movements.
16 Gestational Weeks
The fetal crown-rump length is 12 cm, and the weight is 110 g. By 14 weeks, gender can be determined by experienced observers by inspection of the external genitalia. Eye movements begin at 16 to 18 weeks, coinciding with midbrain maturation.
20 Gestational Weeks
This is the midpoint of pregnancy as estimated from the beginning of the last menses. The fetus now weighs somewhat more than 300 g, and weight increases in a linear manner. From this point onward, the fetus moves about every minute and is active 10 to 30 percent of the time (DiPietro, 2005). The fetal skin has become less transparent, a downy lanugo covers its entire body, and some scalp hair has developed. Cochlear function develops between 22 and 25 weeks, and its maturation continues for six months after delivery.
24 Gestational Weeks
The fetus now weighs approximately 630 g. The skin is characteristically wrinkled, and fat deposition begins. The head is still comparatively large, and eyebrows and eyelashes are usually recognizable. The canalicular period of lung development, during which the bronchi and bronchioles enlarge and alveolar ducts develop, is nearly completed. A fetus born at this time will attempt to breathe, but many will die because the terminal sacs, required for gas exchange, have not yet formed. By 26 weeks, nociceptors are present over all the body, and the neural pain system is developed (Kadic, 2012).
28 Gestational Weeks
The crown-rump length is approximately 25 cm, and the fetus weighs about 1100 g. The thin skin is red and covered with vernix caseosa. The pupillary membrane has just disappeared from the eyes. Isolated eye blinking peaks at 28 weeks. The otherwise normal neonate born at this age has a 90-percent chance of survival without physical or neurological impairment.
32 and 36 Gestational Weeks
At 32 weeks, the fetus has attained a crown-rump length of about 28 cm and a weight of approximately 1800 g. The skin surface is still red and wrinkled. In contrast, by 36 weeks, the fetal crown-rump length averages about 32 cm, and the weight is approximately 2500 g. Because of subcutaneous fat deposition, the body has become more rotund, and the previous wrinkled facial appearance has been lost.
40 Gestational Weeks
This is considered term from the onset of the last menstrual period. The fetus is now fully developed. The average crown-rump length is about 36 cm, and the weight is approximately 3400 g.
PLACENTAL PHYSIOLOGY AND FETAL GROWTH
The placenta is the organ of transfer between mother and fetus. At this maternal-fetal interface, there is transfer of oxygen and nutrients from the mother to the fetus and carbon dioxide and metabolic wastes from fetus to mother. There are no direct communications between fetal blood, which is contained in the fetal capillaries of the chorionic villi, and maternal blood, which remains in the intervillous space. Instead, bidirectional transfer depends on the processes that permit or aid the transport through the syncytiotrophoblast that line chorionic villi.
That said, there are occasional breaks in the chorionic villi, which permit escape of fetal cells into the maternal circulation. This leakage is the mechanism by which some D-negative women become sensitized by the erythrocytes of their D-positive fetus (Chap. 15, p. 306). It can also lead to chimerism from entrance of allogeneic fetal cells, including trophoblast, into maternal blood (Sunami, 2010). These are estimated to range from 1 to 6 cells/mL around midpregnancy, and some are “immortal” (Lissauer, 2007). A clinical corollary is that some maternal autoimmune diseases may be provoked by such chimerism (Bloch, 2011; Boyon, 2011). This is also discussed in Chapter 59 (p. 1168).
The Intervillous Space
Maternal blood within the intervillous space is the primary unit of maternal–fetal transfer. Blood from the maternal spiral arteries directly bathes the trophoblasts. Substances transferred from mother to fetus first enter the intervillous space and are then transported to the syncytiotrophoblast. Thus, the chorionic villi and intervillous space function together as the fetal lung, gastrointestinal tract, and kidney.
Circulation within the intervillous space is described in Chapter 5 (p. 95). Intervillous and uteroplacental blood flow increases throughout the first trimester of normal pregnancies (Mercé, 2009). At term, the residual volume of the intervillous space measures about 140 mL. Before delivery, however, the volume of this space may be twice this value (Aherne, 1966). Uteroplacental blood flow near term has been estimated to be 700 to 900 mL/min, with most of the blood apparently going to the intervillous space.
Active labor contractions reduce blood flow into the intervillous space. The degree of reduction depends on the contraction intensity. Blood pressure within the intervillous space is significantly less than uterine arterial pressure, but somewhat greater than venous pressure. The latter, in turn, varies depending on several factors, including maternal position. When supine, for example, pressure in the lower part of the inferior vena cava is elevated, and consequently, pressure in the uterine and ovarian veins, and in turn in the intervillous space, is increased.
Placental Transfer
Substances that pass from maternal to fetal blood must first traverse the syncytiotrophoblast, then villous stroma, and finally, the fetal capillary wall. Although this histological barrier separates maternal and fetal circulations, it is not a simple physical barrier. First, throughout pregnancy, syncytiotrophoblast actively or passively permits, facilitates, and adjusts the amount and rate of substance transfer to the fetus. The maternal-facing syncytiotrophoblast surface is characterized by a complex microvillous structure. The fetal-facing basal cell membrane is the site of transfer to the intravillous space. Finally, the villous capillaries are an additional site for transport from the intravillous space into fetal blood, or vice versa. In determining the effectiveness of the human placenta as an organ of transfer, at least 10 variables are important, as shown in Table 7-2 and described next.
TABLE 7-2. Variables of Maternal-Fetal Substance Transfer
Maternal plasma concentration and carrier-protein binding of the substance
Maternal blood flow rate through the intervillous space
Trophoblast surface area size available for exchange
Physical trophoblast properties to permit simple diffusion
Trophoblast biochemical machinery for active transport
Substance metabolism by the placenta during transfer
Fetal intervillous capillary surface area size for exchange
Fetal blood concentration of the substance
Specific binding or carrier proteins in the fetal or maternal circulation
Villous capillary blood flow rate
Mechanisms of Transfer
Most substances with a molecular mass less than 500 Da pass readily through placental tissue by simple diffusion. These include oxygen, carbon dioxide, water, most electrolytes, and anesthetic gases (Carter, 2009). Some low-molecular-weight compounds undergo transfer facilitated by syncytiotrophoblast. These are usually those that have low concentrations in maternal plasma but are essential for normal fetal development.
Insulin, steroid hormones, and thyroid hormones cross the placenta, but very slowly. The hormones synthesized in situ in the trophoblasts enter both the maternal and fetal circulations, but not equally (Chap. 5, p. 101). Examples are concentrations of chorionic gonadotropin and placental lactogen, which are much lower in fetal plasma than in maternal plasma. Substances of high molecular weight usually do not traverse the placenta, but there are important exceptions. One is immunoglobulin G—molecular weight 160,000 Da—which is transferred by way of a specific trophoblast receptor-mediated mechanism.
Transfer of Oxygen and Carbon Dioxide
Placental oxygen transfer is blood-flow limited. Using estimated uteroplacental blood flow, Longo (1991) calculated oxygen delivery to be approximately 8 mL O2/min/kg of fetal weight. Normal values for oxygen, carbon dioxide, and pH in fetal blood are presented in Figure 7-7. Because of the continuous passage of oxygen from maternal blood in the intervillous space to the fetus, its oxygen saturation resembles that in maternal capillaries. The average oxygen saturation of intervillous blood is estimated to be 65 to 75 percent, with a partial pressure (Po2) of 30 to 35 mm Hg. The oxygen saturation of umbilical vein blood is similar, but with a somewhat lower oxygen partial pressure.
FIGURE 7-7 Umbilical venous oxygen pressure (Po2) (A); carbon dioxide pressure (Pco2) (B); and pH (C) from cordocentesis performed in fetuses being evaluated for possible intrauterine infections or hemolysis, but who were found to be healthy at birth and appropriately grown. (From Ramsay, 1996, with permission.)
The placenta is highly permeable to carbon dioxide, which traverses the chorionic villus by diffusion more rapidly than oxygen. Near term, the partial pressure of carbon dioxide (Pco2) in the umbilical arteries averages about 50 mm Hg, or approximately 5 mm Hg more than in the maternal intervillous blood. Fetal blood has less affinity for carbon dioxide than does maternal blood, thereby favoring carbon dioxide transfer from fetus to mother. Also, mild maternal hyperventilation results in a fall in Pco2 levels, favoring a transfer of carbon dioxide from the fetal compartment to maternal blood (Chap. 4, p. 63).
Selective Transfer and Facilitated Diffusion
Although simple diffusion is an important method of placental transfer, the trophoblast and chorionic villus unit demonstrate enormous selectivity in transfer. This results in different metabolite concentrations on the two sides of the villus. Importantly, the levels of many substances that are not synthesized by the fetus are several times higher in fetal than in maternal blood. Ascorbic acid is one example. This relatively low-molecular-weight substance might be expected to traverse the placenta by simple diffusion. The concentration of ascorbic acid, however, is two to four times higher in fetal plasma than in maternal plasma (Morriss, 1994). Another example is the unidirectional transfer of iron. Typically, the mother’s plasma iron concentration is much lower than that of her fetus. Even with severe maternal iron deficiency anemia, the fetal hemoglobin mass is normal.
FETAL NUTRITION
Because of the small amount of yolk in the human ovum, growth of the embryo-fetus is dependent on maternal nutrients during the first 2 months. During the first few days after implantation, blastocyst nutrition comes from the interstitial fluid of the endometrium and the surrounding maternal tissue.
Maternal adaptations to store and transfer nutrients to the fetus are discussed in Chapter 4 and summarized here. Three major maternal storage depots—the liver, muscle, and adipose tissue—and the storage-hormone insulin are intimately involved in the metabolism of the nutrients absorbed from the maternal gut.
Insulin secretion is sustained by increased serum levels of glucose and amino acids. The net effect is storage of glucose as glycogen primarily in liver and muscle, retention of some amino acids as protein, and storage of the excess as fat. Storage of maternal fat peaks in the second trimester and then declines as fetal energy demands increase in the third trimester (Pipe, 1979). Interestingly, the placenta appears to act as a nutrient sensor, altering transport based on the maternal supply and environmental stimuli (Fowden, 2006; Jansson, 2006b).
During times of fasting, glucose is released from glycogen, but maternal glycogen stores cannot provide an adequate amount of glucose to meet requirements for maternal energy and fetal growth. Augmentation is provided by cleavage of triacylglycerols, stored in adipose tissue, which result in free fatty acids and activation of lipolysis.
Glucose and Fetal Growth
Although dependent on the mother for nutrition, the fetus also actively participates in providing for its own nutrition. At midpregnancy, fetal glucose concentration is independent of and may exceed maternal levels (Bozzetti, 1988). Glucose is the major nutrient for fetal growth and energy. Logically, mechanisms exist during pregnancy to minimize maternal glucose use so that the limited maternal supply is available to the fetus. Human placental lactogen (hPL), a hormone normally abundant in the mother but not the fetus, is believed to block peripheral uptake and use of glucose, while promoting mobilization and use of free fatty acids by maternal tissues (Chap. 5, p. 104).
Glucose Transport
The transfer of d-glucose across cell membranes is accomplished by a carrier-mediated, stereospecific, nonconcentrating process of facilitated diffusion. There are 14 glucose transport proteins (GLUTs) encoded by the SLC2A gene family and characterized by tissue-specific distribution (Leonce, 2006). GLUT-1 and GLUT-3 primarily facilitate glucose uptake by the placenta and are located in the plasma membrane of the syncytiotrophoblast microvilli (Korgun, 2005). DNA methylation regulates expression of placental GLUT genes, with epigenetic modification across gestation (Novakovic, 2013). GLUT-1 expression is essential for decidualization. It increases as pregnancy advances and is induced by almost all growth factors (Frolova, 2011; Sakata, 1995).
Lactate is a product of glucose metabolism and is also transported across the placenta by facilitated diffusion. By way of cotransport with hydrogen ions, lactate is probably transported as lactic acid.
Glucose and Insulin Role in Fetal Macrosomia
The precise biomolecular events in the pathophysiology of fetal macrosomia are not defined. Nonetheless, it seems clear that fetal hyperinsulinemia is one driving force (Schwartz, 1994). As discussed in Chapter 44 (p. 872), insulin-like growth factor and fibroblast growth factor are important regulators of placental development and function (Forbes, 2010; Giudice, 1995). In addition, recent work has shown that placental corticotropin-releasing hormone (CRH) stimulates trophoblastic production of GLUT-1 and inhibits expression of GLUT-3 through interaction on the CRH-R1 receptor. This suggests a role for CRH in the nutritional regulation of fetal growth and development (Gao, 2012).
Leptin
This polypeptide hormone was originally identified as a product of adipocytes and a regulator of energy homeostasis. It also contributes to angiogenesis, hemopoiesis, osteogenesis, pulmonary maturation, and neuroendocrine, immune, and reproductive functions (Henson, 2006; Maymó, 2009). Leptin is produced by the mother, fetus, and placenta. It is expressed in syncytiotrophoblast and fetal vascular endothelial cells. Of placental production, 5 percent enters fetal circulation, whereas 95 percent is transferred to the mother (Hauguel-de Mouzon, 2006). As a result, the placenta greatly contributes to maternal leptin levels.
Fetal leptin levels begin rising at approximately 34 weeks and are correlated with fetal weight. Abnormal levels have been associated with growth disorders and preeclampsia. Postpartum, leptin levels decline in both the newborn and mother (Grisaru-Granovsky, 2008). Perinatal leptin is associated with the development of metabolic syndromes later in life (Granado, 2012).
Free Fatty Acids and Triglycerides
The newborn has a large proportion of fat, which averages 15 percent of body weight (Kimura, 1991). Thus, late in pregnancy, a substantial part of the substrate transferred to the human fetus is stored as fat. Although maternal obesity affects placental fatty acid uptake, it appears to have no effect on fetal growth (Dube, 2012). Neutral fat in the form of triacylglycerols does not cross the placenta, but glycerol does. There is preferential placental-fetal transfer of long-chain polyunsaturated fatty acids (Gil-Sanchez, 2012). Lipoprotein lipase is present on the maternal but not on the fetal side of the placenta. This arrangement favors hydrolysis of triacylglycerols in the maternal intervillous space while preserving these neutral lipids in fetal blood. Fatty acids transferred to the fetus can be converted to triacylglycerols in the fetal liver.
The placental uptake and use of low-density lipoprotein (LDL) is an alternative mechanism for fetal assimilation of essential fatty acids and amino acids (Chap. 5, p. 109). The LDL binds to specific receptors in the coated-pit regions of the syncytiotrophoblast microvilli. The large—about 250,000 Da—LDL particle is taken up by a process of receptor-mediated endocytosis. The apoprotein and cholesterol esters of LDL are hydrolyzed by lysosomal enzymes in the syncytium to give: (1) cholesterol for progesterone synthesis; (2) free amino acids, including essential amino acids; and (3) essential fatty acids, primarily linoleic acid. Indeed, the concentration of arachidonic acid, which is synthesized from linoleic acid in fetal plasma, is greater than that in maternal plasma. Linoleic acid or arachidonic acid or both must be assimilated from maternal dietary intake.
Amino Acids
The placenta concentrates many amino acids in the syncytiotrophoblast, which are then transferred to the fetal side by diffusion (Lemons, 1979). Based on data from cordocentesis blood samples, the amino acid concentration in umbilical cord plasma is greater than in maternal venous or arterial plasma (Morriss, 1994). Transport system activity is influenced by gestational age and environmental factors. These include heat stress, hypoxia, and under- and overnutrition, as well as hormones such as glucocorticoids, growth hormone, and leptin (Fowden, 2006). Trophoblastic mammalian target of rapamycin complex 1 (mTORC1) regulates placental amino acid transporters and modulates transfer across the placenta (Jansson, 2012). In vivo studies suggest an upregulation of transport for certain amino acids and an increased fetal delivery in women with gestational diabetes associated with fetal overgrowth (Jansson, 2006a).
Proteins
Placental transfer of larger proteins is limited, but there are exceptions. Immunoglobulin G (IgG) crosses the placenta in large amounts via endocytosis and trophoblast Fc receptors. IgG transfer depends on maternal levels of total IgG, gestational age, placental integrity, IgG subclass, and antigen nature (Palmeira, 2012). IgA and IgM of maternal origin are effectively excluded from the fetus (Gitlin, 1972).
Ions and Trace Metals
Iodide transport is clearly attributable to a carrier-mediated, energy-requiring active process. And indeed, the placenta concentrates iodide. The concentrations of zinc in the fetal plasma also are greater than those in maternal plasma. Conversely, copper levels in fetal plasma are less than those in maternal plasma. This fact is of particular interest because important copper-requiring enzymes are necessary for fetal development.
Placental Sequestration of Heavy Metals
The heavy-metal–binding protein metallothionein-1 is expressed in human syncytiotrophoblast. This protein binds and sequesters a host of heavy metals, including zinc, copper, lead, and cadmium. Lead enters the fetal environment at a level 90 percent of maternal concentrations, but placental transfer of cadmium is limited (Kopp, 2012). The most common source of environmental cadmium is cigarette smoke. Cadmium levels in maternal blood and placenta are increased with maternal smoking, but there is no increase in cadmium transfer into the fetus. In the rat, data suggest that cadmium reduces the number of trophoblasts, leading to poor placental growth (Lee, 2009).
Metallothionein also binds and sequesters copper (Cu2+) in placental tissue. This accounts for the low levels of Cu2+ in cord blood (Iyengar, 2001). Several enzymes require Cu2+, and its deficiency results in inadequate collagen cross-linking and, in turn, diminished tensile strength of tissues. This may be important because the concentration of cadmium in amnionic fluid is similar to that in maternal blood. The incidence of preterm membrane rupture is increased in women who smoke. It is possible that cadmium provokes metallothionein synthesis in the amnion. This may cause sequestration of Cu2+, a pseudocopper deficiency, and in turn, a weakened amnion.
Calcium and Phosphorus
These minerals also are actively transported from mother to fetus. Calcium is transferred for fetal skeletal mineralization (Olausson, 2012). A calcium-binding protein is produced in placenta. Parathyroid hormone-related protein (PTH-rP), as the name implies, acts as a surrogate PTH in many systems (Chap. 5, p. 105). PTH is not demonstrable in fetal plasma. However, PTH-rP is present, suggesting that PTH-rP is the fetal parathormone. The expression of PTH-rP in cytotrophoblasts is modulated by the extracellular concentration of Ca2+ (Hellman, 1992). It seems possible, therefore, that PTH-rP synthesized in decidua, placenta, and other fetal tissues is important in fetal Ca2+ transfer and homeostasis.
Vitamins
The concentration of vitamin A (retinol) is greater in fetal than in maternal plasma and is bound to retinol-binding protein and to prealbumin. Retinol-binding protein is transferred from the maternal compartment across the syncytiotrophoblast. The transport of vitamin C—ascorbic acid—from mother to fetus is accomplished by an energy-dependent, carrier-mediated process. The levels of the principal vitamin D—cholecalciferol–metabolites, including 1,25-dihydroxycholecalciferol, are greater in maternal plasma than are those in fetal plasma. The 1β-hydroxylation of 25-hydroxyvitamin D3 is known to take place in placenta and in decidua.
FETAL ORGAN SYSTEM DEVELOPMENT
Amnionic Fluid Formation
In early pregnancy, amnionic fluid is an ultrafiltrate of maternal plasma. By the second trimester, it consists largely of extracellular fluid that diffuses through the fetal skin and thus reflects the composition of fetal plasma (Gilbert, 1993). After 20 weeks, the cornification of fetal skin prevents this diffusion, and amnionic fluid is composed largely of fetal urine. Fetal kidneys start producing urine at 12 weeks, and by 18 weeks, they are producing 7 to 14 mL per day. Fetal urine contains more urea, creatinine, and uric acid than fetal plasma. Amnionic fluid also contains desquamated fetal cells, vernix, lanugo, and various secretions. Because these are hypotonic, the net effect is that amnionic fluid osmolality decreases with advancing gestation. Pulmonary fluid contributes a small proportion of the amnionic volume, and fluid filtering through the placenta accounts for the rest. Aquaporins 8 and 9 play a role in water flow via intramembranous absorption and placental water transfer (Jiang, 2012)
The volume of amnionic fluid at each week is variable (Chap. 11, p. 231). In general, the volume increases by 10 mL per week at 8 weeks and increases to 60 mL per week at 21 weeks, then peaks at 34 weeks (Brace, 1989).
Amnionic fluid serves to cushion the fetus, allowing musculoskeletal development and protecting it from trauma. It also maintains temperature and has a minimal nutritive function. Epidermal growth factor (EGF) and EGF-like growth factors, such as transforming growth factor-β, are present in amnionic fluid. Ingestion of fluid into the gastrointestinal tract and inhalation into the lung may promote growth and differentiation of these tissues. Animal studies have shown that pulmonary hypoplasia can be produced by draining amnionic fluid, by chronically draining pulmonary fluid through the trachea, or by physically preventing the prenatal chest excursions that mimic breathing (Adzick, 1984; Alcorn, 1977). Thus, intrapulmonary fluid formation and, at least as important, the alternating egress and retention of fluid in the lungs by breathing movements are essential to normal pulmonary development.
Cardiovascular System
The fetal circulation is substantially different from that of the adult and functions until birth, when it is required to change dramatically. For example, because fetal blood does not need to enter the pulmonary vasculature to be oxygenated, most of the right ventricular output bypasses the lungs. In addition, the fetal heart chambers work in parallel, not in series, which effectively supplies the brain and heart with more highly oxygenated blood than the rest of the body.
Oxygen and nutrient materials required for fetal growth and maturation are delivered from the placenta by the single umbilical vein (Fig. 7-8). The vein then divides into the ductus venosus and the portal sinus. The ductus venosus is the major branch of the umbilical vein and traverses the liver to enter the inferior vena cava directly. Because it does not supply oxygen to the intervening tissues, it carries well-oxygenated blood directly to the heart. In contrast, the portal sinus carries blood to the hepatic veins primarily on the left side of the liver, and oxygen is extracted. The relatively deoxygenated blood from the liver then flows back into the inferior vena cava, which also receives less oxygenated blood returning from the lower body. Blood flowing to the fetal heart from the inferior vena cava, therefore, consists of an admixture of arterial-like blood that passes directly through the ductus venosus and less well-oxygenated blood that returns from most of the veins below the level of the diaphragm. The oxygen content of blood delivered to the heart from the inferior vena cava is thus lower than that leaving the placenta.
FIGURE 7-8 The intricate nature of the fetal circulation is evident. The degree of blood oxygenation in various vessels differs appreciably from that in the postnatal state. aa = arteries; LA = left atrium; LV = left ventricle; RA = right atrium; RV = right ventricle; v = vein.
In contrast to postnatal life, the ventricles of the fetal heart work in parallel, not in series. Well-oxygenated blood enters the left ventricle, which supplies the heart and brain, and less oxygenated blood enters the right ventricle, which supplies the rest of the body. These two separate circulations are maintained by the right atrium structure, which effectively directs entering blood to either the left atrium or the right ventricle, depending on its oxygen content. This separation of blood according to its oxygen content is aided by the pattern of blood flow in the inferior vena cava. The well-oxygenated blood tends to course along the medial aspect of the inferior vena cava and the less oxygenated blood flows along the lateral vessel wall. This aids their shunting into opposite sides of the heart. Once this blood enters the right atrium, the configuration of the upper interatrial septum—the crista dividens—preferentially shunts the well-oxygenated blood from the medial side of the inferior vena cava through the foramen ovale into the left heart and then to the heart and brain (Dawes, 1962). After these tissues have extracted needed oxygen, the resulting less oxygenated blood returns to the right atrium through the superior vena cava.
The less oxygenated blood coursing along the lateral wall of the inferior vena cava enters the right atrium and is deflected through the tricuspid valve to the right ventricle. The superior vena cava courses inferiorly and anteriorly as it enters the right atrium, ensuring that less well-oxygenated blood returning from the brain and upper body also will be shunted directly to the right ventricle. Similarly, the ostium of the coronary sinus lies just superior to the tricuspid valve so that less oxygenated blood from the heart also returns to the right ventricle. As a result of this blood flow pattern, blood in the right ventricle is 15 to 20 percent less saturated than blood in the left ventricle.
Almost 90 percent of blood exiting the right ventricle is shunted through the ductus arteriosus to the descending aorta. High pulmonary vascular resistance and comparatively lower resistance in the ductus arteriosus and the umbilical–placental vasculature ensure that only about 15 percent of right ventricular output—8 percent of the combined ventricular output—goes to the lungs (Teitel, 1992). Thus, one third of the blood passing through the ductus arteriosus is delivered to the body. The remaining right ventricular output returns to the placenta through the two hypogastric arteries, which distally become the umbilical arteries. In the placenta, this blood picks up oxygen and other nutrients and is recirculated through the umbilical vein.
Circulatory Changes at Birth
After birth, the umbilical vessels, ductus arteriosus, foramen ovale, and ductus venosus normally constrict or collapse. With the functional closure of the ductus arteriosus and the expansion of the lungs, blood leaving the right ventricle preferentially enters the pulmonary vasculature to become oxygenated before it returns to the left heart. Virtually instantaneously, the ventricles, which had worked in parallel in fetal life, now effectively work in series. The more distal portions of the hypogastric arteries, which course from the level of the bladder along the abdominal wall to the umbilical ring and into the cord as the umbilical arteries, undergo atrophy and obliteration within 3 to 4 days after birth. These become the umbilical ligaments, whereas the intraabdominal remnants of the umbilical vein form the ligamentum teres. The ductus venosus constricts by 10 to 96 hours after birth and is anatomically closed by 2 to 3 weeks, resulting in formation of the ligamentum venosum (Clymann, 1981).
Hematological Development
Hemopoiesis
In the early embryo, hemopoiesis is demonstrable first in the yolk sac, followed by the liver and finally bone marrow. The first erythrocytes released into the fetal circulation are nucleated and macrocytic. Mean cell volumes are expressed in femtoliters (fL), and one femtoliter equals one cubic micrometer. The mean cell volume is at least 180 fL in the embryo and decreases to 105 to 115 fL at term. The erythrocytes of aneuploid fetuses generally do not undergo this maturation and maintain high mean cell volumes—130 fL on average (Sipes, 1991). As fetal development progresses, more and more of the circulating erythrocytes are smaller and nonnucleated. With fetal growth, both the blood volume in the common fetoplacental circulation and hemoglobin concentration increase. Hemoglobin content of fetal blood rises to approximately 12 g/dL at midpregnancy and to 18 g/dL at term (Walker, 1953). Because of their large size, fetal erythrocytes have a short life span, which progressively lengthens to approximately 90 days at term (Pearson, 1966). As a consequence, red blood cell production is increased. Reticulocytes are initially present at high levels, but decrease to 4 to 5 percent of the total at term. Fetal erythrocytes differ structurally and metabolically from those of the adult. They are more deformable, which serves to offset their higher viscosity. They also contain several enzymes with appreciably different activities (Smith, 1981).
Erythropoiesis is controlled primarily by fetal erythropoietin because maternal erythropoietin does not cross the placenta. Fetal hormone production is influenced by testosterone, estrogen, prostaglandins, thyroid hormone, and lipoproteins (Stockman, 1992). Serum erythropoietin levels increase with fetal maturity. Although the exact production site is disputed, the fetal liver appears to be an important source until renal production begins. There is a close correlation between the erythropoietin concentration in amnionic fluid and that in umbilical venous blood obtained by cordocentesis. After birth, erythropoietin normally may not be detectable for up to 3 months.
In contrast, platelet production reaches stable levels by mid-pregnancy, although there is some variation across gestation (Fig. 7-9). The fetal and neonatal platelet count is subject to various agents as discussed in Chapter 15 (p. 313).
Figure 7-9 Platelet counts by gestational age obtained the first day of life. Mean values and 5th and 95th percentiles are shown. (Data from Christensen, 2012.)
Fetoplacental Blood Volume
Although precise measurements of human fetoplacental blood volume are lacking, Usher and associates (1963) reported values in term normal newborns to average 78 mL/kg when immediate cord clamping was conducted. Gruenwald (1967) found the fetal blood volume contained in the placenta after prompt cord clamping to average 45 mL/kg of fetal weight. Thus, fetoplacental blood volume at term is approximately 125 mL/kg of fetal weight.
Fetal Hemoglobin
This tetrameric protein is composed of two copies of two different peptide chains, which determine the type of hemoglobin produced. Normal adult hemoglobin A is made of α and β chains. During embryonic and fetal life, various α and β chain precursors are produced. This results in the serial production of several different embryonic hemoglobins. Genes for β-type chains are on chromosome 11 and for α-type chains on chromosome 16. This sequence is shown in Figure 7-10. Each of these genes is turned on and then off during fetal life, until the α and β genes, which direct the production of hemoglobin A, are permanently activated.
FIGURE 7-10 Schematic drawing of the arrangement of the α and β gene precursors on chromosomes 11 and 16 and the hemoglobin types made from them. (Modified after Thompson, 1991.)
The timing of the production of each of these early hemoglobins corresponds to the site of hemoglobin production. Fetal blood is first produced in the yolk sac, where hemoglobins Gower 1, Gower 2, and Portland are made. Erythropoiesis then moves to the liver, where fetal hemoglobin F is produced. When hemopoiesis finally moves to the bone marrow, adult-type hemoglobin A appears in fetal red blood cells and is present in progressively greater amounts as the fetus matures (Pataryas, 1972).
The final adult version of the α chain is produced exclusively by 6 weeks. After this, there are no functional alternative versions. If an α-gene mutation or deletion occurs, there is no alternate α-type chain that could substitute to form functional hemoglobin. In contrast, at least two versions of the β chain—δ and γ—remain in production throughout fetal life and beyond. In the case of a β-gene mutation or deletion, these two other versions of the β chain often continue to be produced, resulting in hemoglobin A2 or hemoglobin F, which substitute for the abnormal or missing hemoglobin.
Genes are turned off by methylation of their control region, which is discussed in Chapter 13 (p. 272). In some situations, methylation does not occur. For example, in newborns of diabetic women, hemoglobin F may persist due to hypomethylation of the γ gene (Perrine, 1988). With sickle-cell anemia, the γ gene remains unmethylated, and large quantities of fetal hemoglobin continue to be produced (Chap. 56, p. 1108). Increased hemoglobin F levels are associated with fewer sickle-cell disease symptoms, and pharmacological modification of these levels by hemoglobin F-inducing drugs is one approach to disease treatment (Trompeter, 2009).
There is a functional difference between hemoglobins A and F. At any given oxygen tension and at identical pH, fetal erythrocytes that contain mostly hemoglobin F bind more oxygen than do those that contain nearly all hemoglobin A (Fig. 47-2, p. 945). This is because hemoglobin A binds 2,3-diphosphoglycerate (2,3-DPG) more avidly than does hemoglobin F, thus lowering the affinity of hemoglobin A for oxygen (De Verdier, 1969). During pregnancy, maternal 2,3-DPG levels are increased, and because fetal erythrocytes have lower concentrations of 2,3-DPG, the latter has increased oxygen affinity.
The amount of hemoglobin F in fetal erythrocytes begins to decrease in the last weeks of pregnancy. At term, approximately three fourths of total hemoglobin is hemoglobin F. During the first 6 to 12 months of life, the hemoglobin F proportion continues to decrease and eventually reaches the low levels found in adult erythrocytes. Glucocorticosteroids mediate the switch from fetal to adult hemoglobin, and the effect is irreversible (Zitnik, 1995).
Coagulation Factors
There are no embryonic forms of the various hemostatic proteins. With the exception of fibrinogen, the fetus starts producing normal, adult-type procoagulant, fibrinolytic, and anticoagulant proteins by 12 weeks. Because they do not cross the placenta, their concentrations at birth are markedly below the levels that develop within a few weeks of life (Corrigan, 1992). In normal neonates, the levels of factors II, VII, IX, X, and XI, as well as those of prekallikrein, protein S, protein C, antithrombin, and plasminogen, are all approximately 50 percent of adult levels. In contrast, levels of factors V, VIII, XIII, and fibrinogen are closer to adult values (Saracco, 2009). Without prophylactic treatment, the vitamin K-dependent coagulation factors usually decrease even further during the first few days after birth. This decline is amplified in breast-fed infants and may lead to newborn hemorrhage (Chap. 33, p. 644).
Fetal fibrinogen, which appears as early as 5 weeks, has the same amino acid composition as adult fibrinogen but has different properties (Klagsbrun, 1988). It forms a less compressible clot, and the fibrin monomer has a lower degree of aggregation (Heimark, 1988). Plasma fibrinogen levels at birth are less than those in nonpregnant adults, however, the protein is functionally more active than adult fibrinogen (Ignjatovic, 2011).
Levels of functional fetal factor XIII—fibrin-stabilizing factor—are significantly reduced compared with those in adults (Henriksson, 1974). Nielsen (1969) described low levels of plasminogen and increased fibrinolytic activity in cord plasma compared with that of maternal plasma. Platelet counts in cord blood are in the normal range for nonpregnant adults (see Fig. 7-9).
Despite this relative reduction in procoagulants, the fetus appears to be protected from hemorrhage because fetal bleeding is rare. Excessive bleeding does not usually occur even after invasive fetal procedures such as cordocentesis. Ney and coworkers (1989) have shown that amnionic fluid thromboplastins and a factor(s) in Wharton jelly combine to aid coagulation at the umbilical cord puncture site.
Various thrombophilias may cause thromboses and pregnancy complications in adults (Chap. 52, p. 1029). If the fetus inherits one of these mutations, thrombosis and infarction can develop in the placenta or in fetal organs. This is usually seen with homozygous inheritance.
Plasma Proteins
Liver enzymes and other plasma proteins are produced by the fetus, and these levels do not correlate with maternal levels (Weiner, 1992). Concentrations of plasma proteins, albumin, lactic dehydrogenase, aspartate aminotransferase, γ-glutamyl transpeptidase, and alanine transferase all increase, whereas prealbumin levels decrease with gestational age (Fryer, 1993). At birth, mean total plasma protein and albumin concentrations in fetal blood are similar to maternal levels (Foley, 1978).
Immunology
Infections in utero have provided an opportunity to examine mechanisms of the fetal immune response. Evidence of immunological competence has been reported as early as 13 weeks (Kohler, 1973; Stabile, 1988). In cord blood at or near term, the average level for most components is approximately half that of the adult values (Adinolfi, 1977).
In the absence of a direct antigenic stimulus such as infection, fetal plasma immunoglobulins consist almost totally of transferred maternal immunoglobulin G (IgG). Thus, antibodies in the newborn are most often reflective of maternal immunological experiences.
Immunoglobulin G
Maternal IgG transport to the fetus begins at approximately 16 weeks and increases thereafter. The bulk of IgG is acquired during the last 4 weeks of pregnancy (Gitlin, 1971). Accordingly, preterm neonates are endowed relatively poorly with protective maternal antibodies. Newborns begin to slowly produce IgG, and adult values are not attained until 3 years of age. In certain situations, the transfer of IgG antibodies from mother to fetus can be harmful rather than protective to the fetus. The classic example is hemolytic disease of the fetus and newborn resulting from D-antigen alloimmunization (Chap. 15, p. 306).
Immunoglobulin M and A
In the adult, production of immunoglobulin M (IgM) in response to an antigenic stimulus is superseded in a week or so predominantly by IgG production. In contrast, very little IgM is produced by normal fetuses, and that produced may include antibody to maternal T lymphocytes (Hayward, 1983). With infection, the IgM response is dominant in the fetus and remains so for weeks to months in the newborn. And because IgM is not transported from the mother, any IgM in the fetus or newborn is that which it produced. Increased levels of IgM are found in newborns with congenital infection such as rubella, cytomegalovirus infection, or toxoplasmosis. Serum IgM levels in umbilical cord blood and identification of specific antibodies may be useful in intrauterine infection diagnosis. In infants, adult levels of IgM are normally attained by age 9 months.
Immunoglobulin A (IgA) ingested in colostrum provides mucosal protection against enteric infections. This role as an immune barrier against infection may explain the small amount of fetal secretory IgA found in amnionic fluid (Quan, 1999).
Lymphocytes and Monocytes
The immune system develops early. B lymphocytes appear in fetal liver by 9 weeks and in blood and spleen by 12 weeks. T lymphocytes begin to leave the thymus at approximately 14 weeks. Despite this, the newborn responds poorly to immunization, and especially poorly to bacterial capsular polysaccharides. This immature response may be due to either deficient response of newborn B cells to polyclonal activators, or lack of T cells that proliferate in response to specific stimuli (Hayward, 1983). In the newborn, monocytes are able to process and present antigen when tested with maternal antigen-specific T cells. DNA methylation patterns are developmentally regulated during monocyte-macrophage differentiation and contribute to the antiinflammatory phenotype in macrophages (Kim, 2012).
Skull
Fetal head size is important because an essential feature of labor is the adaptation between the head and the maternal bony pelvis. The firm skull is composed of two frontal, two parietal, and two temporal bones, along with the upper portion of the occipital bone and the wings of the sphenoid. These bones are separated by membranous spaces termed sutures (Fig. 7-11).
FIGURE 7-11 Fetal head at term showing fontanels and sutures.
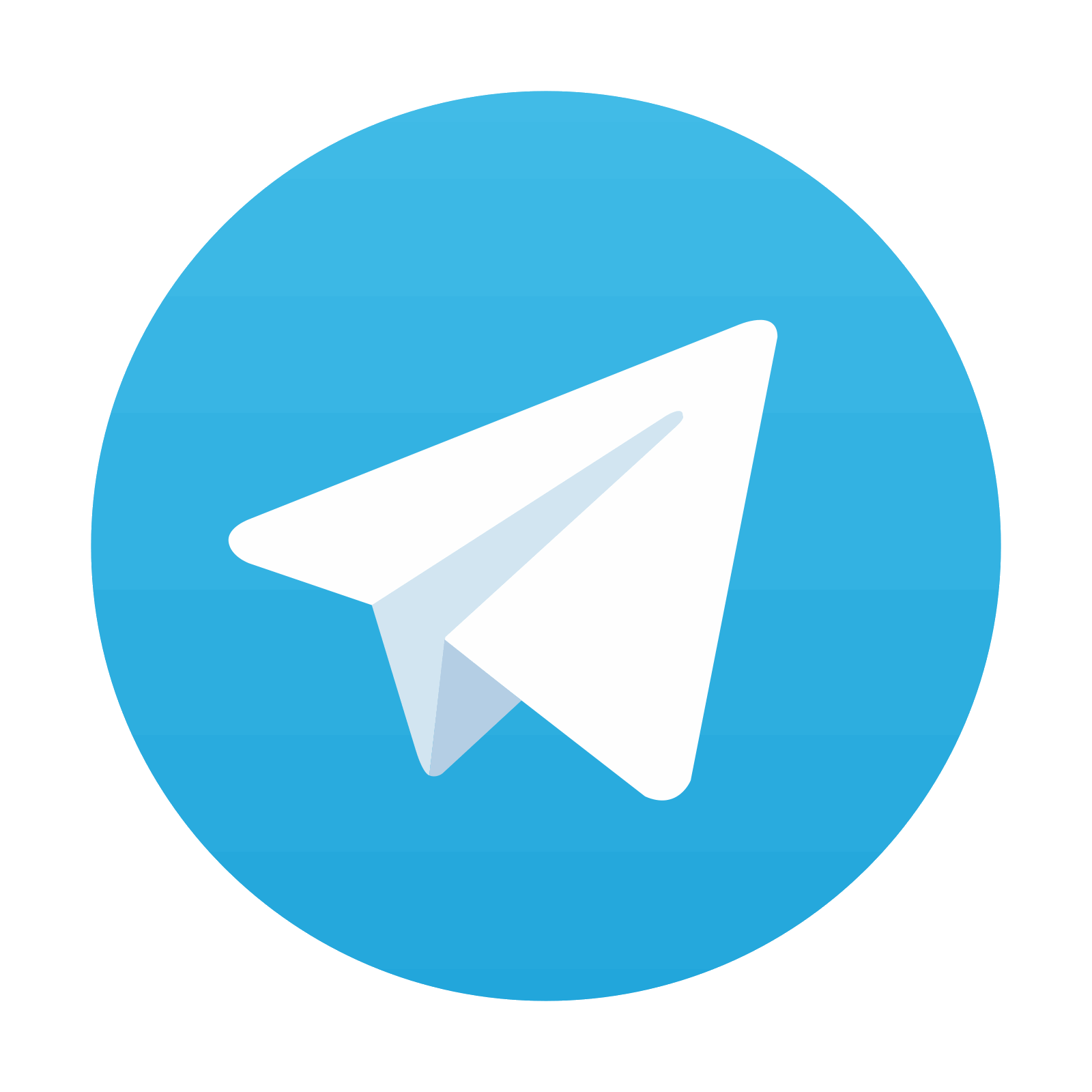
Stay updated, free articles. Join our Telegram channel
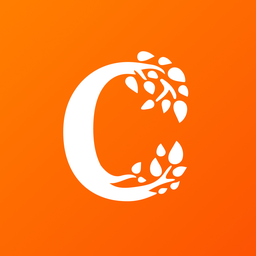
Full access? Get Clinical Tree
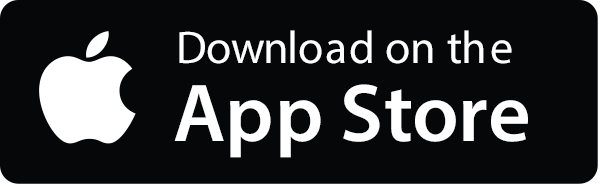
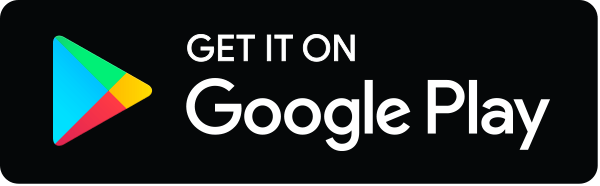