Key Points
- •
The development and structure of the human haemochorial placenta
- •
The development of the uteroplacental and fetoplacental circulations
Introduction
This chapter highlights areas in which developmental placental biology directly impinges on clinical practice. Pregnancies complicated by the pathologies of stillbirth, severe preeclampsia and intrauterine growth restriction (IUGR), especially those that deliver before 34 weeks, are associated with significant gross and microscopic placental pathology (covered in Chapter 9 ). Prenatal diagnosis of pregnancies at risk for these severe complications is now possible by identifying markers of ‘placental insufficiency’ at the 20-week stage. Unfortunately, at the current time, new biomarkers derived from maternal blood samples do not show adequate power of prediction in the first or second trimester. According to the American College of Obstetricians and Gynecologists, ‘current predictive tests for preeclampsia may harm more women than they benefit because of their low positive predictive value (PPV)’ ( https://www.acog.org/Womens-Health/Preeclampsia-and-Hypertension-in-Pregnancy ). This situation underscores the need for a greater understanding of normal placental development and thus a better appreciation of the developmental origins of placental pathology. More detailed information can be found in a review by Dunk and colleagues.
The Placenta at Delivery
A full-term human placenta is a disclike, circular organ commonly inspected in its ‘inverted’ state from the fetal, or chorionic plate, surface. The chorionic plate is a fibrous disc into which the umbilical arteries ramify as chorionic plate vessels, branching in a dichotomous fashion, each penetrating the plate to enter the stem (truncus) of a villous tree, and accompanied by a vein draining oxygenated blood back towards the umbilical vein. The chorionic plate is covered by the amniotic membrane, which is normally glossy and can easily be peeled off. At the placental margin, the chorionic plate thickens to form a ring and continues and fuses with the basal plate to form the chorion laeve, the fetal membranes. The gross structure of a full-term placenta is illustrated in cross section in Fig. 7.1 .

Macroscopic abnormalities, such as succenturiate lobes, velamentous cord insertion or circumvallate margin (in which the ring is undergrown by villous trees and the intervillous space and the membranes insert medial to the edge of the chorionic plate) are identified from this aspect and occur in approximately 10% of cases at term. In isolation these abnormalities have weak associations with low birth weight or preeclampsia and may therefore be ignored if other aspects of placentation are normal. Nevertheless, experience in second trimester ultrasonographic examination of the placenta is increasing, with rare but clinically relevant problems, such as vasa praevia, being identified by ultrasound resulting in dramatic improvements in perinatal survival.
The fetal surface of the placenta may also be inspected at delivery for evidence of intraamniotic infection, such as cloudy yellow malodorous discolouration, or chronic staining by meconium, when clinically relevant. Successful diagnosis of chorioamnionitis at delivery is facilitated by collection of fluid or pus (for gas chromatography), a membrane roll (from rupture point to edge of placenta) and a sample of umbilical cord into sterile containers before transport of the placenta to the pathology department. Cloudy nodular discolourations of the amnion, termed amnion nodosum , are indicative of prolonged rupture of the membranes and oligohydramnios.
The maternal surface of the placenta (referred to as the basal plate) is an artificial surface in that delivery of the ‘placenta’ requires the organ to be cleaved from the uterine wall through the basal plate (see Fig. 7.1 ). In this sense, part of the trophoblast remains undelivered in the placental bed, normally regressing over the ensuing weeks. The basal plate is a therefore heterogeneous mixture of trophoblastic and decidual cells, embedded in large amounts of extracellular debris, fibrinoid and blood clot. The basal surface is divided by a system of grooves into 10 to 40 elevated areas referred to as the maternal lobes of the placenta. These correspond approximately to the underlying arrangement of villous trees with three or four trees per lobe in the centre; small lobes at the periphery of the placenta are generally occupied only by a single villous tree. Only the latter correspond to what has been described as a placentome : a villous tree together with its surrounding part of the intervillous space and its corresponding uteroplacental vessels. Terms such as ‘fetal placenta’ for the chorionic plate (including villous trees) and ‘maternal placental’ for the basal plate (including uteroplacental vessels invaded by trophoblast) are tempting from a clinical perspective, as will be discussed in relation to Doppler studies. However, because it is impossible to physically separate the fetal and maternal cellular constituents, this concept must be viewed with caution.
Many academic departments take an active interest in placental genetics, structure and function as they relate to development, pathology and the new science of perinatal programming. Sampling of the placenta at delivery is thus an increasingly important task. Because the organ is inherently heterogeneous, a strategy of systematic random block sampling, used in studies that use stereology methods, is preferred. When the focus is on vascular structure and villous development, the best approach is to immediately clamp the cord root (to prevent fetoplacental vascular collapse) and allow the organ to fix for several days in formaldehyde. However, because both mRNA’s and proteins are rapidly degraded after delivery, especially in the metabolically active villous trophoblast, when studies at a protein, molecular, or ultrastructural level are undertaken, a better approach is to open the placenta at random sites from the basal plate to excise samples of villous tissue that can be divided and rapidly frozen (for protein extraction) or placed into RNA fixative.
Sampling of the placental bed is far more challenging than that of the delivered placenta. Nevertheless, the introduction of punch biopsy of the placental bed now permits several samples to be taken, including samples from women after vaginal deliveries and earlier gestation miscarriages.
Haemochorial Placental Blood Flow
The human placenta is termed haemochorial because it provides direct contact between maternal blood and the chorionic (fetal) villi in the intervillous space (see Fig. 7.1 ). Maternal blood leaves the openings of the transformed spiral arteries and circulates around the villi. Some villi anchor the villous trees to the basal plate, but the bulk of the term placenta comprises trees of gas-exchanging terminal villi floating in maternal blood. The classic injection studies of spiral arteries by Wigglesworth indicated that a majority of the 60 to 70 villous trees at term, branching from the chorionic plate, are maternally perfused from their centres, thus creating hollow-centred structures. This concept was substantiated by Schuhmann’s description of the 50 to 100 maternal arterial inlets as being located near the centres of the villous trees. The 50 to 200 maternal venous outlets per placenta at term are thought to be arranged around the periphery of the villous trees such that each villous tree is perfused in a centrifugal manner. The radioangiographic studies conducted in humans support the placentome concept which can now be imaged using colour-flow Doppler imaging to study intraplacental blood flow relationships. The maternal arterial jet flows rapidly upwards through the central cavity, dispersing in a centrifugal manner to perfuse the surrounding well developed and more densely packed villi of mature intermediate and terminal type (see later). Short-term reduced intervillous perfusion of a placentome results in temporary hypoxia because fetal perfusion continues to remove oxygen bound to fetal haemoglobin. In response, the stem villous arterioles constrict to reduce the rate of removal of oxygen until intervillous oxygen tension equilibrates again. In this way, the individual placentomes self-regulate to maximise maternal–fetal exchange. Persistent lack of intervillous perfusion can cause stem villous arterial constriction or thrombosis. However, the central portions of the placenta have the largest placentomes and the most-transformed spiral arteries, so central vascular pathology is therefore rare in normal pregnancy. By contrast, spiral artery thrombosis and villous infarction are often found at the margins of the placental disc after a term delivery, with no apparent ill effects.
It is the uniquely invasive properties of the extravillous trophoblast (EVT) that transform the uterine spiral arteries, which result in the haemochorial arrangement that characterises human placentation. The process of invasion is precisely controlled, and it is thought that the disordered EVT proliferation and migration, in combination with the placental vascular pathology, relates to major clinical conditions such as preeclampsia and IUGR (inadequate invasion) or placenta percreta (excessive invasion) and is discussed in detail in Chapter 9 . Please see the online video files associated with Chapter 9 for more information.
Early Stages of Placental Development
Placental development begins with blastocyst attachment to the uterine wall. At this stage, the first extraembryonic cell lineage differentiates and is termed the trophoblast . Signals from the embryo (inner cell mass), including fibroblast growth factor 4 (FGF4), expand the population of trophoblast stem (TS) cells that are capable of differentiating along both the extravillous and villous pathways of development. Considerable knowledge of trophoblast differentiation has been obtained using transgenic mice and mouse TS cells and is summarised in Knott and Paul 2004. Blastocyst symmetry is directed by the inner cell mass because only those trophoblast cells overlying the inner cell mass make direct contact with the uterine epithelium ( Fig. 7.2A ). Abnormal orientation of these structures likely causes abnormalities in the site of umbilical cord insertion into the placental disc and are more common in pregnancies arising from in vitro fertilisation (IVF).

The prelacunar stage of development ( Fig. 7.2B ) is characterised by the formation of an outer shell of syncytiotrophoblast that is distinct from the later villous syncytiotrophoblast by being able to penetrate the uterine epithelium and embed the conceptus in the uterine stroma. The more proximal trophoblast cell population is referred to as cytotrophoblast and is positioned between the syncytiotrophoblast and embryoblast. The cytotrophoblast layer is assumed to be a multipotent stem cell population capable of subsequently producing each type of trophoblast, as in mice. Around day 14 postconception, the conceptus is fully embedded within uterine tissues, and the syncytiotrophoblast starts to develop fluid-filled spaces termed lacunae ( Fig. 7.2C ). The lacunae gradually coalesce to form one large intervillous space of the placenta. This lacunar stage results in the formation of syncytiotrophoblast columns, referred to as trabeculae , which reach from the embryonic side of the placenta to the maternal decidual tissues. The development of the lacunae and the establishment of the intervillous space compartmentalise the growing placenta as follows:
- •
The site of attachment, comprising anchoring villi and the basal plate
- •
The lacunar spaces, forming the intervillous space
- •
Branches that derive from the trabeculae develop into floating villi
- •
The embryonic side develops into the chorionic plate
Further invasion of the maternal tissues by placental trophoblast cells is necessary so as to transform the spiral arteries of the uteroplacental circulation as well as the uterine glands. The first step is the streaming of cytotrophoblast cells down the centre of the syncytiotrophoblast trabeculae, creating a new lineage of extravillous cytotrophoblast that is in direct contact with maternal tissues beyond the initial wave of syncytiotrophoblast invasion ( Fig. 7.2D–F ). Cytotrophoblast at the tips of the trabeculae, their maternal ends now referred to as anchoring villi, form trophoblast cell columns. The proximal cells proliferate as the source of all subsequent subtypes of invasive EVT cells. Initially, the invasion of maternal decidual tissues by cytotrophoblast begins in the connective tissue (interstitial EVT) followed by the walls of the spiral arteries and uterine veins (endovascular trophoblast cells) or the epithelium of uterine glands.
Trophoblast invasion into maternal tissues is not restricted to the process of implantation and early placentation but is a continuous process throughout pregnancy, serving a number of purposes. The trophoblastic cell columns at the base of the anchoring villi provide the cellular sources for this invasive process. The cell columns do not contain stroma because they were not excavated by mesenchyme during formation of the villous trees. The cytotrophoblast cells composing these cell columns, together with trophoblast cells in the placental bed, basal plate, chorionic plate and membranes, are collectively described as EVT cells.
The trophoblastic cell columns resting on the basal plate should be viewed as a rapidly proliferating zone from which EVT cells migrate continuously into maternal tissues ( Fig. 7.3 ). However, as soon as the EVT cells leave this proliferative zone, they leave the cell division cycle and change to an invasive phenotype. Their pattern of integrin expression, secretion of proteolytic enzymes and production of extracellular matrix (ECM) proteins is strikingly similar to that of malignant tumour cells. Fortunately, they differ in one fundamental aspect: they do not proliferate during invasion. This is one of the reasons why the depth of invasion into maternal tissues is limited. If deportation into the maternal circulation occurs, metastatic growth is impossible because these cells have lost their generative potency.

Invasion by EVT serves three very different purposes, namely adhesion of the placenta to the uterine wall, adaptation of uterine glands to enable histotrophic nutrition during the first trimester of pregnancy and adaptation of uteroplacental arteries and veins, enabling haemochorial nutrition to meet the fetal requirements later in gestation.
Phenotypes of Extravillous Trophoblast
Among the invasive trophoblast cells, a subset of cells secretes huge amounts of ECM (composed of laminins, collagen IV, fibronectins, vitronectin and heparan sulphate) known as matrix-type fibrinoid into which they are embedded. The EVT cells adhere to the ECM via the surface expression of molecules known as integrins. Likewise, the endometrial stromal cells adhere via similar mechanisms; root-like projections of EVT, together with its associated matrix, penetrate into the inner third of the myometrium, thereby ensuring anchoring of the placenta. Adhesion of the placenta by the gluelike ECM depends on the viability of the EVT cells expressing integrins; in this sense, it is reversible at delivery. This anchoring process is essential; otherwise, entry of maternal blood into the intervillous space at high velocity would shear off the whole placenta.
Along the invasive pathway through the decidual interstitium, EVT cells show morphologically and functionally different phenotypes. These different phenotypes display a varying behaviour regarding contact to maternal cells, secretion of matrix and invasiveness. At present, the molecular basis of these different phenotypes is not known, although it has been described in the related mouse placenta. Such knowledge may in the future help understanding of the pathways that prevent this physiologic process from occurring in certain specific diseases, such as abruption (premature separation), preeclampsia and IUGR.
In a normal intrauterine pregnancy, EVT cells that are invading the decidual interstitium can be subdivided into three morphologically and functionally different subtypes.
Large Polygonal Cells
This subtype of large polygonal EVT cells corresponds to the well described former X-cells. Compared with the subset of small spindle-shaped cells, the relative number of this subtype increases from 45% at weeks 9 to 12 to 69% at weeks 16 to 24 and makes up about 89% in weeks 31 to 39. Hence, this subtype is the prevailing phenotype of EVT at the time of delivery. These cells are evenly distributed throughout the basal plate as well as along the route of invasion reaching the inner third of the myometrium. Morphologically, these cells are large, polygonal, uninucleated EVT cells displaying big, irregularly shaped, intensely staining nuclei. No other subtype of all trophoblast cells displays a stronger immunoreactivity for cytokeratin 7 than these large polygonal cells. At the same time, they are always immunonegative for proliferation markers such as anti-Ki 67.
These cells secrete the typical ECM of EVT, the matrix-type fibrinoid. This basement membrane-like ECM comprises three different patches of matrix molecules: (i) collagen IV and laminin, (ii) an amorphous ground substance containing heparan sulphate and vitronectin, and (iii) fibronectins and fibrillin embedded in the same amorphous ground substance. The large cells fix themselves within their self-secreted matrix by expression and exposition of the respective integrins, such as α5/β1, α1/β1 and α-v/β3/5 integrins, reviewed by Harris and colleagues. These cells organise themselves in clusters, which are usually void of maternal tissue components but filled with matrix-type fibrinoid. These features do not support the classical thinking that the large polygonal cells (‘X-cells’) are highly invasive cells. Rather, it appears as if this subtype of interstitial EVT may have a function in fixing and adhering the placenta to the uterine wall by secreting matrix-type fibrinoid, which has been termed the ‘trophoblast glue’.
Small Spindle-Shaped Extravillous Trophoblast Cells
This subtype of the interstitial trophoblast is also negative for proliferation markers and only moderately immunoreactive for cytokeratin 7 and can be found from the transitional zone of trophoblastic cell columns reaching the inner third of the myometrium. Hence, this subtype shares the spatial distribution pattern with the subtype of the large polygonal cells. Opposing the large polygonal subtype, this small subtype displays a decrease in numbers towards term from 55% in the first trimester to 31% in the second trimester reaching only about 11% at term. Structurally, this subtype is characterised by elongated, partly filiform cell bodies mostly oriented radially to the uterine wall containing small ovoid nuclei. This small subtype usually forms loosely arranged arrays of cells, surrounded by only very little matrix.
There are only a few descriptions of these small spindle-shaped trophoblast cells so far, which may be because these small, usually filiform cells easily escape the investigators’ attention, the reason being that they are rarely represented in one section in full length. The small cells only secrete little amounts of self-secreted matrix, which is mostly composed of cellular and oncofetal isoforms of fibronectin. At the same time, these cells only express ‘interstitial’ integrins such as α5/β1 and α-v-integrins. The predominant expression of ‘interstitial’ integrins in combination with the expression of oncofetal fibronectins is an essential mechanism of trophoblast invasiveness. Interactions between α5/β1 integrins and fibronectins are crucial for trophoblast invasion.
In the past decade, an integrin switch has been described during the course of trophoblast invasion. In light of the data presented, this phenomenon could be explained as follows: The small subtype of cells expresses only ‘interstitial’ integrins and prevails in deeper zones of the invasive pathway because this phenotype is really invasive. The large subtype additionally expresses ‘epithelial’ integrins. Therefore the integrin switch may relate to the terminal differentiation of the motile spindle-shaped form to the nonmotile polygonal trophoblast cells. This process occurs over the course of gestation and may account for the increasing percentage of polygonal trophoblast cells and corresponding decrease in spindle-shaped trophoblast cells.
Multinucleated Giant Cells
This subtype prevails in the depth of the placental bed at the border between decidua and myometrium and does not show any proliferative activity. A distinct difference to the earlier mentioned subtypes is that these cells contain more than 1 and up to 10 irregularly shaped nuclei of varying size, leading to a much larger volume with a diameter ranging between 50 and 100 μm. These multinucleated cells are either immunonegative for cytokeratin 7 or show few spots of reactivity and thus may easily be missed during superficial inspection of immunohistochemical sections of the placental bed. Although a fusion event of such cells has never been observed, it is generally accepted that fusion of interstitial EVT cells does occur.
Regulation of Trophoblast Invasion
The invasive EVT cells finally reach the inner third of the myometrium, where they can be found between the layers of smooth muscle cells. Arrest at this stage is crucial so that pathological placental invasion does not occur. Some insight into the mechanisms that permit further trophoblast invasion into the uterus has come from studies in placenta accreta specimens.
Hypotheses Favouring Extrinsic Factors:
- •
Trigger gradient: EVT cells need a trigger to be invasive. This trigger is derived from the mesenchymal stroma of anchoring villi. Cell invasion is therefore limited by a requirement for this diffusible factor.
- •
Cellular interaction: Cells within the myometrium (smooth muscle cells, specific immune cells) or endometrium may arrest invasion.
Hypotheses Favouring Intrinsic Factors and Programs:
- •
Apoptosis: Programmed cell death occurs in EVT cells. The rates of apoptosis differ significantly among studies because of technical and sampling limitations. Therefore at present, the role of apoptosis as a critical regulator of trophoblast invasion is uncertain.
- •
Polyploidisation: The subtype of large polygonal trophoblast cells is probably differentiated from the small spindle-shaped cells by polyploidisation. This leads to the noninvasive phenotype that secretes ample matrix and anchors the placenta to its implantation site.
- •
Syncytial fusion: It is generally accepted that the multinucleated giant cells derive from syncytial fusion of their mononucleated counterparts. The noninvasive multinucleated cells accumulate close to the border between decidua and myometrium and may act as a trap for the trophoblast cells that try to trespass into deeper layers. Cell–cell fusion likely occurs via expression and interaction of the fusogenic proteins syncytin with its receptor ASCT-2 and connexin 43. These terminally differentiated multinucleated structures may participate in the maternal recognition and adaptation to pregnancy.
The invasive nature of haemochorial placentation is precisely regulated under normal circumstances; when it occurs to excess, EVT cells may invade deeply into (accreta) or through (percreta) the uterine wall, such that physiological separation of the placenta can no longer occur. Invasive placentation is more common in a scarred uterus from multiple previous caesarean sections and in pregnancies after successful endoscopic surgery for Asherman syndrome (uterine adhesion from patchy loss of endometrium).
Conversely, a failure of this invasive anchoring process predisposes to premature placental separation (abruption), either during the antepartum period or during labour. These important conditions underscore the importance of understanding how EVT invasion and production of an adhesive matrix is disturbed in these conditions.
Placental Perfusion During Embryogenesis
During implantation, the expanding and invading early syncytiotrophoblast initially comes into contact with the superficial capillary system of the decidua underneath the uterine epithelium. Capillaries may leak maternal erythrocytes into the lacunar spaces of the placenta, resulting in the presence of a few erythrocytes in the early intervillous space. However, the predominant observation is that the stromal spiral arteries and arterioles are blocked by EVT, and no arterial connections are made between the maternal circulation and the primitive intervillous space. This apparent lack of intervillous blood perfusion during the first trimester of pregnancy has been shown by transcervical endoscopic observations and Doppler ultrasound of the intervillous space. During embryogenesis, the intervillous space is filled with a clear fluid known as uterine milk comprising filtered maternal plasma and uterine gland secretion products rich in lipids, nutrients and growth factors crucial for placental and embryonic development.
Occlusion of maternal arteries during implantation is designed to facilitate embryogenesis in a low-oxygen environment below 20 mm Hg until week 10 of gestation and has the following advantages :
- •
Reduction of the amount of free radicals to protect the embryo from teratogenesis during this critical phase of tissue and organ development.
- •
Mammalian cells grow much faster under low oxygen compared with higher oxygen tensions. Embryo development is characterised by rapid cell division, and thus low oxygen is ideal to generate an environment to achieve and maintain a high level of cell divisions.
- •
Connection between placental and embryonic vessels is not fully established before week 7 of gestation. Hence, there is no need to perfuse the placenta with maternal blood to feed the embryo before this time.
- •
Perfusion of maternal plasma without blood cells may protect the early villous syncytiotrophoblast from direct contact with circulating maternal immune cells.
Transformation of the Uteroplacental Arteries
Starting from the interstitial route of invasion, a subset of EVT cells penetrates the walls of uterine veins and spiral arteries. This subset of invasive EVT cells is termed the endovascular trophoblast . The endovascular trophoblast cells intravasate from the decidual interstitium into the lumen of the arteries, where they may migrate inside the arterial lumen along the arterial wall and later may even extravasate to focally reenter the walls of the spiral arteries. The major role of the endovascular trophoblast is to transform the distal spiral arteries into dilated segments that facilitate increased uteroplacental blood flow. The transformation of spiral arteries is divided into three stages ( Fig. 7.4 ).
- 1.
Maternal factors induce the first changes of uterine spiral arteries ( Fig. 7.4A ) very early during pregnancy, still in the absence of any invading trophoblast in their vicinity, the vessels begin to dilate via a disorganisation of vascular smooth muscle cells and altered endothelial cell morphology. Recently, a number of studies have shown that the maternal immune cells of the decidua are responsible for these changes. They accumulate around the decidual arterioles and play an active role in the early stages of transformation of these vessels through the secretion of growth factors and matrix metalloproteinases to degrade the ECM ( Fig. 7.4B ).
- 2.
At the same time, the EVT begins to separate from the anchoring cell column and invade the decidua interstitially. There is some evidence to suggest that the interstitial EVT can penetrate the maternal vasculature and contribute to the degradation of the vessel wall ( Fig. 7.4C ). Where anchoring cell columns form in the vicinity of a decidual vessel, endovascular EVT cells accumulate in a plug in the proximal portion of the decidual arteries and prevent early entry of maternal blood ( Fig. 7.4D ).
- 3.
Endovascular trophoblast then migrates down the lumen of the vessel, relining the wall as they go, resulting in remodelling of the uteroplacental arteries ( Fig. 7.4E ). As they reline the vessel, they begin to express endothelial markers such as platelet endothelial cell adhesion molecule-1 (PECAM-1) and α-vβ3 integrin. This further dilation of the arteries results in lumen diameters several times greater than the original. The reduced activity of smooth muscle cells and the loss of elastic fibres are clear indications of the disruptive properties of the decidual leukocytes and endovascular trophoblast in the vessel media.
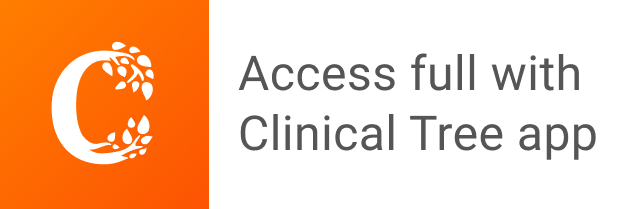