Pathogenesis
Acute respiratory failure, defined as the inability of the respiratory system to adequately deliver oxygen or remove carbon dioxide, causes significant morbidity and mortality in critically ill children, accounting for approximately 50% of deaths in children younger than 1 year of age. Anatomic and developmental differences place infants at higher risk than adults for respiratory failure. An infant’s thoracic cage is more compliant than that of the adult or older child, allowing a greater tendency toward alveolar collapse. The intercostal muscles are poorly developed and unable to achieve the “bucket-handle” motion characteristic of adult breathing, and the diaphragm is shorter and relatively flat with fewer type I muscle fibers, making it less effective and more easily fatigued. The infant’s airways are smaller in caliber than those in older children and adults, resulting in greater resistance to inspiratory and expiratory airflow and greater susceptibility to occlusion by mucus plugging and mucosal edema. Compared with adults, the alveoli of children are also smaller and have less collateral ventilation, again resulting in a greater tendency to collapse and develop atelectasis. Finally, young infants may have an especially reactive pulmonary vascular bed, impaired immune system, or residual effects from prematurity, all of which increase the risk of respiratory failure.
Respiratory failure can be due to inadequate oxygenation (hypoxemic respiratory failure) or inadequate ventilation (hypercapnic respiratory failure) or both. Hypoxemic respiratory failure occurs in three situations: (1) V/Q mismatch, which occurs when blood flows to parts of the lung that are inadequately ventilated, or when ventilated areas of the lung are inadequately perfused; (2) diffusion defects, caused by thickened alveolar membranes or excessive interstitial fluid at the alveolar-capillary junction; and (3) intrapulmonary shunt, which occurs when structural anomalies in the lung allow blood to flow through the lung without participating in gas exchange. Hypercapnic respiratory failure results from impaired alveolar ventilation, due to conditions such as increased dead space ventilation, reduced respiratory drive due to CNS dysfunction or over-sedation, or neuromuscular disorders (Table 14–1).
Table 14–1. Types of respiratory failure.
Clinical Findings
The clinical findings in respiratory failure are the result of hypoxemia, hypercapnia, and arterial pH changes. Common features of respiratory failure are summarized in Table 14–2. These features are not consistently clinically obvious, and most of them have nonrespiratory causes as well. As a result, a strictly clinical assessment of respiratory failure is not always reliable, and clinical findings of respiratory failure should be supplemented by laboratory data such as blood gas analysis.
Table 14–2. Clinical features of respiratory failure.
Noninvasive Monitoring and Blood Gas Analysis
The adequacy of oxygenation and ventilation can be measured both noninvasively and through blood gas analysis. Arterial oxygen saturation (Sao2) can be measured continuously and noninvasively by pulse oximetry, a technique that should be used in the assessment and treatment of all patients with potential or actual respiratory failure. Pulse oximetry readings, however, become markedly less accurate in patients with saturations below approximately 80%, poor skin perfusion, or significant movement. In addition, pulse oximetry can be dangerously inaccurate in certain clinical settings such as carbon monoxide poisoning or methemoglobinemia. End-tidal CO2 (ETCO2) monitoring provides a continuous noninvasive means of assessing the adequacy of ventilation. The ETCO2 level closely approximates the alveolar CO2 level (Paco2), which should equal the arterial CO2 level (Paco2) because carbon dioxide diffuses freely across the alveolar-capillary barrier. While most accurate in the intubated patient, this technique can also be used in extubated patients with the proper equipment. Though useful for following trends in ventilation, ETCO2 monitoring is also susceptible to significant error, particularly in patients with rapid, shallow breathing or increased dead space ventilation.
Given the limitations of these noninvasive techniques, arterial blood gas (ABG) analysis remains the gold standard for assessment of acute respiratory failure. ABGs provide measurements of the patient’s acid-base status (with a measured pH and calculated bicarbonate level) as well as Pao2 and Paco2 levels. Although measurement of capillary or venous blood gases may provide some reassurance regarding the adequacy of ventilation and can be useful for following trends, they yield virtually no useful information regarding oxygenation and may generate highly misleading information about the ventilatory status of patients who have poor perfusion or who had difficult blood draws. As a result, ABG analysis is important for all patients with suspected respiratory failure, particularly those with abnormal venous or capillary gases.
Knowing the ABG values and the inspired oxygen concentration also enables one to calculate the alveolar-arterial oxygen difference (A–aDO2, or A–a gradient). The A–a gradient is less than 15 mm Hg under normal conditions, though it widens with increasing inspired oxygen concentrations to about 100 mm Hg in normal patients breathing 100% oxygen. This number has prognostic value in severe hypoxemic respiratory failure, with A–a gradients over 400 mm Hg being strongly associated with mortality. Diffusion impairment, shunts, and V/Q mismatch all increase the A–a gradient. In addition to the calculation of the A–a gradient, assessment of intrapulmonary shunting (the percentage of pulmonary blood flow that passes through nonventilated areas of the lung) may be helpful. Normal individuals have less than a 5% physiologic shunt from bronchial, coronary, and thebesian (cardiac intramural) circulations. Shunt fractions greater than 15% usually indicate the need for aggressive respiratory support. When intrapulmonary shunt reaches 50% of pulmonary blood flow, Pao2 does not significantly increase regardless of the amount of supplemental oxygen used. Calculation of the shunt fraction requires a pulmonary arterial catheter for measurement of mixed venous blood gases; for patients without pulmonary artery catheters, the A–a gradient is a good surrogate measure of intrapulmonary shunting.
Modes of Respiratory Support
Patients with severe hypoxemia, hypoventilation, or apnea require immediate assistance with bag and mask ventilation until the airway is successfully intubated and controlled mechanical ventilation can be provided. Assisted ventilation with a bag and mask can generally be maintained for some time with a mask of the proper size, but gastric distention, emesis leading to aspiration of gastric contents, and inadequate tidal volumes leading to atelectasis are possible complications. In those patients not requiring immediate intubation, a variety of modalities can be used to provide respiratory support, including supplemental oxygen, heated high flow nasal cannula (HHFNC), and noninvasive ventilation (NIV) with continuous positive airway pressure (CPAP) or bilevel positive airway pressure (BIPAP).
Supplemental oxygen with a nasal cannula or oxygen mask may be adequate to treat patients with mild respiratory insufficiency (Table 14–3). Patients with hypoventilation and diffusion defects respond better to supplemental oxygen than patients with significant shunts or V/Q mismatch. Heated high-flow nasal cannula (HHFNC) devices utilize a nasal cannula for delivery of heated and humidified oxygen mixtures at high flow rates not normally tolerated with cooler drier air. This approach is increasingly being used in infants and young children but is also well tolerated in older patients. Generally, flow rates of greater than 2 L/min in infants and greater than 4 L/min in children are considered high flow. HHFNC use has been studied in children with bronchiolitis and appears to be well tolerated, potentially decreasing the need for intubation by providing some amount of airway positive pressure. HHFNC should be considered in patients who need more respiratory support than a simple low-flow nasal cannula, but if the patient is not improving on HHFNC after 60–90 minutes, additional escalation of care may be warranted. Furthermore, although HHFNC provides some amount of positive pressure, the exact amount of positive pressure cannot be accurately determined from the flow rate. If a patient needs further escalation of care, the device used should be capable of more reliably delivering a fixed amount of positive pressure, such as CPAP or BIPAP.
Table 14–3. Supplemental oxygen therapy.
Noninvasive ventilation (NIV) refers to the administration of mechanical ventilatory support without using an invasive artificial airway (endotracheal tube or tracheostomy tube). The use of NIV has become an integral tool in the management of both acute and chronic respiratory failure. NIV can be used to avoid endotracheal intubation for milder cases of respiratory failure and as a bridge to extubation in mechanically ventilated patients with marginal lung function and respiratory mechanics. NIV devices provide positive pressure breathing through a variety of interfaces (mouth piece or nasal, face, or helmet mask) and using a variety of ventilatory modes, including continuous positive airway pressure (CPAP), bilevel positive airway pressure (BiPAP), volume ventilation, and pressure support. Ventilators dedicated to NIV exist, and many standard ventilators are capable of providing support through a mask with the appropriate adapters. Most current NIV ventilator models incorporate oxygen blenders for precise delivery of the fraction of inspired oxygen (Fio2).
Continuous positive airway pressure (CPAP) refers to the constant application of airway pressure, usually in the range of 5–8 cm H2O, and seeks to improve work of breathing, ventilation, and oxygenation by maintaining the functional residual capacity (FRC) of the lungs. Bilevel positive airway pressure (BIPAP) functions similarly but cycles between a higher inspiratory pressure (IPAP) and a lower expiratory pressure (EPAP). The additional inspiratory support in this mode improves tidal volume and ventilation in patients who are breathing shallowly and can improve oxygenation by providing a higher mean airway pressure. Typical initial settings would place the IPAP at 10–14 cm H2O and the EPAP at 6–8 cm H2O. The IPAP can then be titrated upward to achieve adequate tidal volumes, usually in the range of 5–7 mL/kg, and to reduce the patient’s work of breathing and respiratory rate toward the normal range. EPAP and delivered oxygen concentration can be adjusted upward on the basis of pulse oximetry to achieve adequate oxygenation. Serial blood gas measurements are essential to monitor the response to therapy and to guide further ventilator adjustments.
Successful application of NIV requires careful patient selection. The best candidates are patients in the recovery phases of their illness or those with primarily hypercapnic respiratory failure, such as patients with muscular dystrophies or other forms of neuromuscular weakness. Patients suffering from coma, impaired respiratory drive, an inability to protect their airway, or cardiac or respiratory arrest are not candidates for NIV. Controversy still exists regarding the safety of NIV as an initial strategy in patients with acute hypoxemic respiratory failure, but in general NIV appears to be well tolerated in many children with this condition and it may decrease the risk for intubation. These patients should be closely monitored, however, as NIV may mask symptoms of underlying disease progression, making eventual intubation more precarious. In patients with severe respiratory failure or those who are worsening on NIV, endotracheal intubation should not be delayed.
For patients with respiratory failure not responding adequately to noninvasive support, endotracheal intubation and the initiation of mechanical ventilation can be life-saving. Safe placement of an endotracheal tube in infants and children requires experienced personnel and appropriate equipment at the bedside, including supplemental oxygen, correctly sized mask and bag oral airways, and endotracheal tubes, and suction catheters. The patient should first be positioned properly to facilitate air exchange while supplemental oxygen is given. The sniffing position is used in infants. Head extension with jaw thrust is used in older children without neck injuries. If obstructed by secretions or vomitus, the airway must be cleared by suction. When not obstructed and properly positioned, the airway should be patent and easily visualized, allowing the placement of an oral or nasopharyngeal endotracheal tube of the correct size. Patients with normal airway anatomy may be intubated under intravenous (IV) anesthesia by experienced personnel (Table 14–4). Endotracheal intubation of patients with significant upper airway obstruction (eg, patients with croup, epiglottitis, foreign bodies, or subglottic stenosis) or mediastinal masses should be approached with extreme caution; minimal sedation should be used and paralytic agents should be strictly avoided unless trained airway specialists decide otherwise.
Table 14–4. Drugs commonly used for controlled endotracheal intubation.
The size of the endotracheal tube (ETT) is of critical importance in pediatrics. An inappropriately large endotracheal tube can cause pressure necrosis in the subglottic region, potentially leading to scarring and stenosis requiring surgical repair. An inappropriately small endotracheal tube can result in inadequate pulmonary toilet and excessive air leak around the endotracheal tube, making adequate ventilation and oxygenation difficult. Two useful methods for calculating the correct size of endotracheal tube for a child are (1) measuring the child’s height with a Broselow Tape and then reading the corresponding endotracheal tube size on the tape, or (2) in children older than age 2 years, choosing a tube size using the formula ETT size = (16 + age in years) ÷ 4. Approximate proper insertion depth (in cm) as measured at the teeth can be estimated by tripling the size of the ETT. Either cuffed or uncuffed tubes are appropriate; preference should be given for a cuffed tube in patients with significant lung disease likely to have poor lung compliance. Correct placement of the endotracheal tube should be confirmed by auscultation for the presence of equal bilateral breath sounds and by the use of a colorimetric filter (pH-sensitive indicator that changes from purple to yellow when exposed to carbon dioxide) to detect carbon dioxide. An assessment of air leakage around the endotracheal tube is an important measure of the appropriateness of endotracheal tube size. An audible leak noted at pressures of 15–20 cm H2O indicates acceptable ETT size, though higher leak pressures are acceptable in patients who have poor lung compliance and as a result require higher airway pressures to effectively ventilate and oxygenate. A chest radiograph is necessary for final assessment of endotracheal tube placement. A correctly positioned ETT will terminate in the mid-trachea between the thoracic inlet and the carina, at approximately the level of the second thoracic vertebrae.
CONVENTIONAL MECHANICAL VENTILATION
Indications
The principal indications for institution of mechanical ventilation are acute and chronic respiratory failure or an airway rendered unstable either by illness, injury, or treatment with sedating medications. Examples of these conditions include pneumonia, sepsis, trauma, neuromuscular disease, and procedural sedation. The goals of mechanical ventilation are to facilitate the movement of gas into and out of the lungs (ventilation) and to improve oxygen uptake into the bloodstream (oxygenation). While life-saving in many situations, positive pressure ventilation can also be harmful. As a result, mechanical ventilation strategies must be adapted to achieve these goals in a way that minimizes further injury to the lung. The overriding principles of this “lung protective ventilation strategy” are to safely recruit under-inflated lung, sustain lung volume, minimize phasic overdistention, and decrease lung inflammation. This strategy requires adjustment of ventilator settings with an understanding of the difference between the gas exchange that is permissible and that which is normal or optimal.
Modes of Mechanical Ventilation
The parameters used to control the delivery of mechanical ventilation breaths are known as the trigger, cycle, control, and limit variables. The trigger variable describes how breaths are initiated, either by the patient or by the ventilator. The most common triggers are patient effort, sensed as a drop in return pressure or gas flow to the ventilator, and time. A newer trigger method, neurally adjusted ventilatory assist (NAVA), measures the electrical activity of the diaphragm via an esophageal catheter in order to adjust the ventilator breaths to meet the patient’s neural activity. While NAVA holds promise as a means to improving patient-ventilator synchrony and facilitating ventilator weaning, its ideal role in clinical practice remains to be determined. The cycle variable describes how the inspiratory phase is terminated, either by the patient or by the ventilator. Most ventilator modes cycle according to a set inspiratory time (I-time) although flow-cycled modes can be used in spontaneously breathing patients. The control variable determines whether the ventilator delivers a specific tidal volume (volume-controlled modes) or a specific pressure (pressure-controlled modes). Limit variables are parameters whose magnitude is constrained during inspiration in order to prevent excessive pressure or volume from being delivered by the ventilator.
Breathing during mechanical ventilation can be classified as spontaneous or mandatory. The patient controls the timing and size of spontaneous breaths. The ventilator controls the timing and/or size of mandatory breaths, independent of patient activity. In addition, the breathing pattern provided by the ventilator can be set to one of three configurations. In continuous mandatory ventilation (CMV), the ventilator determines the size and duration of all breaths. In intermittent mandatory ventilation (IMV), the ventilator delivers mandatory breaths but additional spontaneous breaths between and during mandatory breaths are allowed. In continuous spontaneous ventilation (CSV), the patient initiates and controls all breaths but the ventilator assists those efforts.
A ventilator mode consists of a specific control variable (pressure or volume), a specific pattern of breathing (CMV, IMV, or CSV), and a specific set of phase variables (trigger, limit, and cycle). Initiation of breaths and the length of exhalation are controlled by setting the respiratory rate. In time-cycled modes of ventilation, the inspiratory time (I-time) determines the length of inspiration and when to allow exhalation. Most modern ventilators can deliver either a pressure-targeted or a volume-targeted breath in several manners. In synchronized intermittent mandatory ventilation (SIMV), the ventilator delivers breaths in an IMV pattern but the machine breaths are synchronized with the patient’s efforts. If the patient does not make adequate respiratory efforts to trigger the ventilator, the machine delivers a mandatory breath at a preset time interval. In pressure support ventilation, the patient’s own efforts are assisted by the delivery of gas flow to achieve a targeted peak airway pressure. Pressure support ventilation allows the patient to determine the rate and pattern of breaths (CSV breathing pattern), thus improving patient comfort and decreasing the work of breathing. The most commonly used mode of ventilation in most PICUs is synchronized intermittent mandatory ventilation with pressure support (SIMV + PS), a mixed mode allowing pressure-supported breaths between the synchronized machine breaths.
One of the ongoing controversies in critical care medicine surrounds the relative roles of volume- vs pressure-controlled modes of ventilation. In pressure-controlled ventilation, air flow begins at the start of the inspiratory cycle and continues until a preset airway pressure is reached. That airway pressure is then maintained until the end of the set I-time, when the exhalation valve on the ventilator opens and gas exits into the machine. With this mode of ventilation, changes in the compliance of the respiratory system will lead to fluctuations in the actual tidal volume delivered to the patient. The advantage of pressure-targeted ventilation lies primarily in the avoidance of high airway pressures that might cause barotrauma or worsen lung injury. The main disadvantage of pressure-controlled ventilation is the possibility of delivering either inadequate or excessive tidal volumes during periods of changing lung compliance. In volume-controlled ventilation, the machine delivers a set tidal volume to the patient. Changes in lung compliance will lead to fluctuations in the peak airway pressure generated by the breath. The main advantage of volume ventilation is more reliable delivery of the desired tidal volume and thus better control of ventilation. More reliable tidal volume delivery may also help prevent atelectasis due to hypoventilation. Disadvantages of volume ventilation include the risk of barotrauma from excessive airway pressures and difficulties overcoming leaks in the ventilator circuit. In either pressure-or volume-controlled modes, alarm limits can be set in order to restrict changes in either tidal volume or airway pressure with changing lung compliance; interpreting those alarms and adjusting the ventilator require the ICU clinician to understand the ventilator mode in use.
Finally, in any mode of ventilation, the minimum distending pressure applied to the lung during the respiratory cycle is determined by setting the positive end-expiratory pressure (PEEP). All mechanical ventilators open their expiratory limbs at the end of inspiration allowing gas release until a preset pressure is achieved; this is the PEEP value. PEEP helps to prevent the end-expiratory collapse of open lung units, thus preventing atelectasis and shunting. In disease states such as pulmonary edema, pneumonia, or ARDS, a higher PEEP (10–15 cm H2O) may increase the patient’s functional residual capacity, helping to keep open previously collapsed alveoli and improve oxygenation. High levels of PEEP may also cause complications such as gas trapping and CO2 retention, barotrauma with resultant air leaks, and decreased central venous return leading to declines in cardiac output or increases in intracranial pressure (ICP).
Setting and Adjusting the Ventilator
When initiating volume-controlled modes of ventilation, the ICU clinician sets a tidal volume, I-time, rate, and level of PEEP. A typical initial tidal volume is 6–10 mL/kg, as long as that volume does not cause excessive airway pressures (> 30 cm H2O). The I-time is typically set at 1 second or 33% of the respiratory cycle, whichever is shorter. Rate can be adjusted to patient comfort and blood gas measurements, but generally patients starting on mechanical ventilation require full support at least initially with a rate of 20–30 breaths/min. Pressure-controlled ventilation is set in a similar fashion, although the adequacy of the inspiratory pressure is assessed by observing the patient’s chest rise and by measuring the delivered tidal volume. Typically, patients without lung disease require peak inspiratory pressures of 15–20 cm H2O, while patients with respiratory illnesses may require 20–30 cm H2O pressure to provide adequate ventilation. In general, PEEP should be set at 5 cm H2O initially and titrated up to maintain adequate oxygenation at acceptable inspired oxygen concentrations (< 60%–65%) while watching carefully for adverse effects on systemic hemodynamics.
Ventilated patients require careful monitoring for the efficacy of gas exchange, including respiratory rate and activity, chest wall movement, and quality of breath sounds. Oxygenation should be measured by ABGs and by continuous pulse oximetry. Ventilation should be assessed by blood gas analysis and by noninvasive means, such as transcutaneous monitoring or ETCO2 sampling. Transcutaneous PO2 or PCO2 measurements are most useful with younger patients who have good skin perfusion, but they become problematic in patients with poorly perfusion, anasarca, or obesity. ETCO2 monitoring involves placing a gas-sampling port on the endotracheal tube and analyzing expired gas for CO2. This technique is more valuable for patients with large tidal volumes, lower respiratory rates, and without significant leaks around the endotracheal tube. In practice, ETCO2 values may differ significantly (usually lower) from measured Paco2 values and thus are most useful for following trends in ventilation, for early recognition of occluded or malpositioned endotracheal tubes, and for assessing the adequacy of chest compressions during CPR. Frequent, preferably continuous, systemic blood pressure monitoring is also necessary for patients ventilated with high PEEP levels, given the risk of adverse hemodynamic effects.
Ventilator settings can be adjusted to optimize both ventilation (Paco2) and oxygenation (Pao2). Ventilation is most closely associated with the delivered minute volume, or the tidal volume multiplied by the respiratory rate. As a result, abnormal Paco2 values can be most effectively addressed by changes in the respiratory rate or the tidal volume. Increased respiratory rate or tidal volume should increase minute volume and thus decrease Paco2 levels; decreases in respiratory rate or tidal volume should act in the opposite fashion. In some circumstances, additional adjustments may also be necessary. For example, for patients with disease characterized by extensive alveolar collapse, increasing PEEP may improve ventilation by helping to keep open previously collapsed lung units. Also, for patients with disease characterized by significant airway obstruction, decreases in respiratory rate may allow more time for exhalation and improve ventilation despite an apparent decrease in the minute volume provided.
The variables most closely associated with oxygenation are the inspired oxygen concentration and the mean airway pressure (MAP) during the respiratory cycle. Increases in inspired oxygen concentration will generally increase arterial oxygenation, unless right-to-left intracardiac or intrapulmonary shunting is a significant component of the patient’s illness. Concentrations of inspired oxygen above 60%–65%, however, may lead to hyperoxic lung injury. For patients requiring those levels of oxygen or higher to maintain adequate arterial saturations, increases in MAP should be considered as a means to recruit underinflated lung units. MAP is affected by PEEP, peak inspiratory pressure, and I-time. Increases in any one of those factors will increase MAP and should improve arterial oxygenation. It is important to bear in mind, however, that increases in MAP may also lead to decreases in cardiac output, primarily by decreasing venous return to the heart. In this circumstance, raising MAP may increase arterial oxygenation, but actually compromise oxygen delivery to the tissues. For patients with severe hypoxemic respiratory failure, these tradeoffs highlight the need for careful monitoring by experienced personnel.
Supportive Care of the Mechanically Ventilated Patient
Patients undergoing mechanical ventilation require meticulous supportive care. Mechanical ventilation is often frightening and uncomfortable for critically ill children. In order to reduce dyssynchrony with the ventilator and impaired gas exchange, careful attention must be directed toward optimizing patient comfort and decreasing anxiety. Sedative-anxiolytics are typically provided as intermittent doses of benzodiazepines, with or without opioids. Some patients respond better to the steady state of sedation provided by continuous infusion of these agents, although oversedation of the ventilated patient may lead to longer duration of ventilation, difficulty with weaning from the ventilator, and other complications. It is beneficial to use standardized assessments of sedation level and target the minimum sedation level necessary to maintain patient comfort and adequate gas exchange.
For patients with severe respiratory illness, even small physical movements can compromise gas exchange. In such cases, muscle paralysis may facilitate oxygenation and ventilation. Nondepolarizing neuromuscular blocking agents are most commonly used for this purpose, given as intermittent doses or as continuous infusions. When muscle relaxants are given, extra care must be taken to ensure that levels of sedation are adequate, as paralytics will mask many of the usual signs of patient discomfort. In addition, ventilator support may need to be increased to compensate for the elimination of patient respiratory effort.
Mechanically ventilated patients can often be fed enterally with the use of nasogastric feeding tubes. However, reflux aspiration leading to ventilator-associated pneumonia can be a concern. In patients where reflux or emesis is a major concern, transpyloric feeding or parenteral nutrition should be considered.
Ventilator-associated pneumonia (VAP) is a significant complication of mechanical ventilation, leading to longer ICU stays and increased hospital costs. As a result, many local and national quality improvement initiatives have focused on minimizing the risks of VAP. These preventative measures include proper hand-washing, elevation of the head of the bed to 30 degrees to prevent reflux, frequent turning of the patient, proper oral care, the use of closed suction circuits on all ventilated patients and avoidance of breaking the closed suction system, sedation protocols to minimize sedation administration, and daily assessment of extubation readiness.
Mechanical ventilation should be weaned and discontinued as soon as safely possible. Extubation failure rates in mechanically ventilated children have been estimated between 4% and 20%. Considerable effort has been devoted to identifying predictors of extubation readiness and success. Unfortunately, the available literature does not clearly support any specific weaning protocol or extubation readiness test. Successful extubation requires adequate gas exchange, adequate respiratory muscle strength, and the ability to protect the airway. If those conditions can be met, most practitioners as a test of extubation readiness will perform a trial of spontaneous breathing in which the patient, while remaining intubated, breathes either without assistance (through a t-piece) or with a low level of pressure support (through the ventilator) for a defined period of time, usually 1–2 hours. The patient is observed carefully for signs of rapid shallow breathing or worsened gas exchange during this trial, and if neither is observed, the patient can generally be safely extubated.
Troubleshooting
Troubleshooting a sudden deterioration in the mechanically ventilated patient should begin with determining whether the endotracheal tube is still in place using direct laryngoscopy and/or ETCO2 measurements. Determine whether the ETT is patent and in the correct position by attempting to pass a suction catheter and by obtaining a chest x-ray if necessary. If the ETT is patent and correctly positioned, the next step is to determine whether any changes in the physical examination—such as poor or unequal chest rise, or absent or unequal breath sounds—suggest atelectasis, bronchospasm, pneumothorax, or pneumonia. Next, determine whether hemodynamic deterioration could be underlying acute respiratory compromise (shock or sepsis). If the problem cannot be readily identified, take the patient off the ventilator and begin manual ventilation by hand-bagging while the ventilator is checked for malfunction. Hand-bagging the patient can also determine the root of the problem if it lies within the patient and can help determine the next ventilator adjustments.
High Frequency Oscillatory Ventilation
High frequency oscillatory ventilation (HFOV) is an alternative mode of mechanical ventilation in which the ventilator provides very small, very rapid tidal volumes at high rates. Respiratory rates used during oscillatory ventilation typically range from 5 to 10 Hz (rates of 300–600 breaths/min) in most PICU patients. This mode of ventilation has been used successfully in neonates, older pediatric patients, and adults, although recent work has suggested that HFOV use may be associated with worse outcomes in adults with ARDS. HFOV is most widely used in severe, diffuse lung diseases, such as ARDS, which require high MAP to maintain lung expansion and oxygenation. Diseases characterized by significant heterogeneity or extensive gas trapping often respond too poorly to HFOV, although reports do exist of successful HFOV use in asthma. The advantage of HFOV is that high levels of MAP can be achieved without high peak inspiratory pressures or large tidal volumes, thus theoretically protecting the lung from ventilator-induced lung injury. Disadvantages of HFOV include general poor tolerance by patients who are not heavily sedated or paralyzed, the risk of cardiovascular compromise due to high MAP, and the risk of gas-trapping and barotrauma in patients with highly heterogeneous lung disease. Although HFOV clearly can be useful as a rescue mode for selected patients, it remains unclear whether HFOV provides any benefit compared with carefully managed conventional modes of ventilation.
Extracorporeal Membrane Oxygenation
Extracorporeal membrane oxygenation (ECMO) has been used as a rescue therapy to support pediatric patients with severe respiratory failure who have not improved with less invasive therapies. ECMO circuits generally consist of a membrane oxygenator, a heater, and a pump. Central venous blood from the patient is directed out of the body, oxygenated, warmed and returned back to the patient. ECMO can be provided in two major modes: venoarterial (VA) and venovenous (VV). VA ECMO bypasses the lungs and the heart, thus supporting both the cardiovascular and respiratory systems, and requires cannulation of a large central artery and vein. VV ECMO utilizes central venous cannulation to provide extracorporeal oxygenation and carbon dioxide removal, thus augmenting the function of the patient’s lungs, but the patient’s own cardiac output is required to provide systemic oxygen delivery. VV ECMO use has increased over the past 15 years and provides the advantage of a reduced risk of systemic and, particularly, cerebral emboli. Patients with moderate hemodynamic compromise prior to ECMO initiation can also experience improvements in circulatory status on VV ECMO, likely due to the improvements in acid base status, oxygenation, and decreased intrathoracic pressures that can be achieved with ECMO. ECMO is indicated for patients with reversible cardiovascular and/or respiratory failure and is not recommended in patients with severe neurologic compromise or who is in the terminal stages of a lethal condition. Despite an increase in the complexity of patients placed on ECMO, survival has remained acceptable over the past 2 decades. According to recent registry data, 57% of pediatric respiratory failure patients who are supported with ECMO survive, and survival rates are even better for ECMO patients with a diagnosis of viral pneumonia (especially due to respiratory syncytial virus) and without significant co-morbidities. Of note, in both neonatal and adult randomized controlled trials, patients with severe respiratory failure who were referred to an ECMO center for consideration of ECMO had improved survival, even though not all patients were actually placed on ECMO. These results emphasize the importance of early referral to experienced centers if ECMO is to be considered.
Determining the optimal time to consider ECMO initiation is one of the most challenging aspects of using this technology. Survival appears equally good for most indications with mechanical ventilation for up to 14 days prior to ECMO initiation. Patients placed on ECMO later in the course of their illness or with prolonged ECMO runs (> 14 days) may have worse outcomes. Protocols to improve secretion clearance and lung recruitment have been described and should be considered to hasten lung recovery and shorten ECMO runs.
While ECMO remains a viable therapy for selected patients with severe respiratory failure, serious complications such as CNS injury, hemorrhage, renal insufficiency, infection, and complications of immobility do occur, and each patient should be carefully evaluated by experienced personnel in order to choose the optimal timing and mode of ECMO support.
Carroll CL et al: Emergent endotracheal intubations in children: be careful if it’s late when you intubate. Pediatr Crit Care Med 2010 May;11(3):343–348 [PMID: 20464775].
Dohna-Schwake C et al: Non-invasive ventilation on a pediatric intensive care unit: feasibility, efficacy, and predictors of success. Pediatric Pulmonology 2011;46(11):1114–1120.
Khemani RG, Newth CJ: The design of future pediatric mechanical ventilation trials for acute lung injury. Am J Respir Crit Care Med 2010 Dec 15;182(12):1465–1474 [PMID: 20732987].
Kissoon N, Rimensberger PC, Bohn D: Ventilation strategies and adjunctive therapy in severe lung disease. Pediatr Clin North Am 2008;55(3):709–733, xii [PMID: 18501762].
Lee JH et al: Use of high flow nasal cannula in critically ill infants, children, and adults: a critical review of the literature. Intensive Care Med 2013;39(2):247–257.
Nava S et al: Non-invasive ventilation in acute respiratory failure. Lancet 2009 Jul 18;374(9685):250–259 [PMID: 19616722].
Newth CJ et al: Eunice Shriver Kennedy National Institute of Child Health and Human Development Collaborative Pediatric Critical Care Research Network: weaning and extubation readiness in pediatric patients. Pediatr Crit Care Med 2009 Jan;10(1):1–11 [PMID: 19057432].
Peak GJ et al: Efficacy and economic assessment of conventional ventilatory support versus extracorporeal membrane oxygenation for severe adult respiratory failure (CESAR): a multicentre randomised controlled trial. Lancet 2009 Oct 17;374(9698):1351–1363.
Santillanes G, Guasche-Hill M: Pediatric airway management. Emerg Med Clin North Am 2008 Nov;26(4):961–975 [PMID: 19059095].
Zabrocki LA et al: Extracorporeal membrane oxygenation for pediatric respiratory failure: survival and predictors of mortality. Crit Care Med 2011 Feb;39(2):364–370.
MAJOR RESPIRATORY DISEASES IN THE PEDIATRIC ICU
ACUTE RESPIRATORY DISTRESS SYNDROME
ARDS is a syndrome of acute respiratory failure characterized by increased pulmonary capillary permeability resulting in bilateral diffuse alveolar infiltrates on chest radiography, decreased lung compliance, and hypoxemia that is usually refractory to supplemental oxygen alone. Mortality rates for pediatric ARDS have fluctuated over time, depending on the criteria used to diagnose the disease, the presence of important coexisting conditions, and the quality and consistency of supportive care provided in the ICU. Mortality rates as high as 60%–75% were reported in the 1980s and early 1990s. Since that time there has been a trend toward decreased mortality in pediatric ARDS, ranging from 8% to 40%, though mortality among immunocompromised patients still approaches 60%. Across all subpopulations of pediatric ARDS patients, nonpulmonary organ failure remains an important cause of mortality.
Current consensus diagnostic criteria for ARDS include (1) acute onset; (2) bilateral pulmonary infiltrates on chest radiograph; (3) pulmonary artery occlusion pressure (PAOP) ≤ 18 mm Hg or no clinical evidence of left atrial hypertension; and (4) severe hypoxemia in which the ratio of the arterial oxygen level (Pao2) to inspired oxygen concentration (Fio2) is ≤ 300 while receiving a PEEP of at least five via mechanical ventilation. When the Pao2:Fio2 ratio is between 200 and 300, the case is defined as mild ARDS, between 100 and 200 is moderate ARDS, and under 100 is severe ARDS. While these criteria remain controversial because of their lack of specificity, they helped to usher in a new era of clinical research that has contributed greatly to what we now know about the pathophysiology ARDS and factors influencing its outcomes in children.
Presentation and Pathophysiology
ARDS may be precipitated by a variety of insults (Table 14–5). Pneumonia and sepsis account for the majority of ARDS cases in children. Despite the diversity of potential causes, the clinical presentation is remarkably similar in most cases. ARDS can be divided roughly into four clinical phases (Table 14–6). In the earliest phase, the patient may have dyspnea and tachypnea with a relatively normal Pao2 and hyperventilation-induced respiratory alkalosis. No significant abnormalities are noted on physical or radiologic examination of the chest. Experimental studies suggest that neutrophils accumulate in the lungs at this stage and that their products damage lung endothelium.
Table 14–5. ARDS risk factors.
Table 14–6. Pathophysiologic changes of acute respiratory distress syndrome.
Over the next few hours, hypoxemia worsens and respiratory distress becomes clinically apparent, with cyanosis, tachycardia, irritability, and dyspnea. Early radiographic changes include the appearance of increasingly confluent alveolar infiltrates initially appearing in dependent lung fields, in a pattern suggestive of pulmonary edema. Proteinaceous exudates into the alveolar space and direct injury to type II alveolar pneumocytes cause surfactant inactivation and deficiency. As a result, the injured lung requires high inflation pressures to achieve lung opening, and increased positive end expiratory pressure (PEEP) to maintain end expiratory volume.
Injury to the alveolar type II cell also reduces the capacity for alveolar fluid clearance. Under normal conditions, sodium is taken up from the alveolar space by channels on the apical surface of type II cells and then actively transported across the basolateral cell membrane into the interstitial space. This process creates a gradient for the passive movement of water across the alveolar epithelium and back into the interstitium. In ARDS, this mechanism becomes overwhelmed as direct lung injury depopulates the alveolar epithelium, creating conditions that favor alveolar fluid accumulation. Pulmonary hypertension, reduced lung compliance, and increased airways resistance are also commonly observed in ARDS. Clinical studies suggest that airways resistance may be increased in up to 50% of patients with ARDS, likely as a result of airway damage or inflammation-induced bronchospasm, although this increased resistance is only rarely clinically important.
Computer tomography (CT) studies of adult patients in the acute phases of ARDS demonstrate a heterogeneous pattern of lung involvement. The most dependent lung regions remain consolidated throughout the respiratory cycle and can only be recruited using exceedingly high inflation pressures. The most nondependent regions are overinflated throughout the respiratory cycle. Between these two zones lies a region that is either normally inflated or repetitively cycles between inflation and collapse. Attempts to improve oxygenation by recruiting the collapsed dependent lung regions occur at the expense of damaging nondependent regions by hyperinflation. This process, termed volutrauma, incites a potent inflammatory response that is capable of worsening nonpulmonary organ dysfunction. Even in normal lungs, ventilation with large tidal volumes and low positive end-expiratory pressure (PEEP) levels can produce a lung injury that is histologically indistinguishable from ARDS. This phenomenon is called ventilator-induced lung injury. Taken together, these findings suggest that mechanical injury from positive pressure ventilation is superimposed on the initial insult and is an integral part of the pathogenesis of ARDS. Appreciation of this phenomenon has prompted a shift toward ventilating ARDS patients with smaller tidal volumes and a tolerance for the relative hypercarbia that typically ensues. Published evidence currently supports using PEEP levels sufficient to stabilize those alveoli with tendency to collapse at end-expiration but below a threshold level that would overdistend nondependent lung regions at end-inspiration. Volutrauma is then mitigated by tidal volume reduction or peak pressure limitation. This approach is termed the “open-lung strategy” of mechanical ventilation.
The subacute phase of ARDS (2–10 days after lung injury) is characterized by type II pneumocyte and fibroblast proliferation in the interstitium of the lung. This results in decreased lung volumes and signs of consolidation that are noted clinically and radiographically. Worsening of the hypoxemia with an increasing shunt fraction occurs, as well as a further decrease in lung compliance. Some patients develop an accelerated fibrosing alveolitis. The mechanisms responsible for these changes are unclear. Current investigation centers on the role of growth and differentiation factors, such as transforming growth factor-β and platelet-derived growth factor released by resident and nonresident lung cells, such as alveolar macrophages, mast cells, neutrophils, alveolar type II cells, and fibroblasts. During the chronic phase of ARDS (10–14 days after lung injury), fibrosis, emphysema, and pulmonary vascular obliteration occur. During this phase of the illness, oxygenation defects generally improve, and the lung becomes more fragile and susceptible to barotrauma. Air leak is common among patients ventilated with high airway pressures at this late stage. Also, patients have increased dead space and difficulties with ventilation are common. Airway compliance remains low because of ongoing pulmonary fibrosis and insufficient surfactant production.
Secondary infections are common in the subacute and chronic phases of ARDS and can impact clinical outcomes. The mechanisms responsible for increased host susceptibility to infection during this phase are not well understood. Mortality in the late phase of ARDS can exceed 80%. Death is usually caused by multiorgan failure and systemic hemodynamic instability rather than by hypoxemia.
Treatment
Contemporary ventilator management of ARDS is directed at protecting vulnerable lung regions from cyclic alveolar collapse at end expiration and protecting overinflated lung regions from hyperinflation at end inspiration. The actual mode of ventilation (eg, volume limited vs pressure limited) employed for ARDS is ultimately not as important as limiting phasic alveolar stretch and stabilizing lung units that are prone to repetitive end expiratory collapse. Over a decade ago, a landmark multicenter trial established that adult ARDS patients who were ventilated using a 6 mL/kg (ideal body weight) tidal volume had a 22% mortality reduction and fewer extrapulmonary organ failures relative to those randomized to receive tidal volumes of 12 mL/kg. The trial also demonstrated a greater reduction in plasma levels of pro-inflammatory cytokines among those in the lower tidal volume group, a finding suggesting that appropriate ventilator strategies can actually reduce the systemic inflammatory response. Although this trial has never been replicated in pediatric patients, application of these same management principles has gained widespread acceptance among pediatric ICU clinicians.
Given the large body of evidence supporting the benefits of low tidal volume ventilation, we suggest that mechanical ventilation of pediatric ARDS patients be initiated using a tidal volume of 6–8 mL/kg (ideal body weight), combined with PEEP sufficient to produce target arterial saturations (≥ 88%–90%) using an Fio2 of ≤ 0.6. In general, this can be accomplished by incremental increases in PEEP until adequate oxygenation is achieved or until a limiting side effect of the PEEP is reached. Whenever escalating mechanical ventilator settings, clinicians should minimize the endotracheal tube cuff leak (if possible), ensure an appropriate plane of patient sedation, and optimize the ventilation to perfusion relationship by verifying that the patient’s intravascular volume status is appropriate. Permissive hypercapnia should be used unless a clear contraindication exists (eg, increased intracranial pressure). If adequate ventilation cannot be achieved (pH remains below 7.25 due to hypercapnia), the ventilator rate can then be increased, provided the patient has time to adequately exhale before the next breath. Subsequently, tidal volume can then be increased as necessary toward 8 mL/kg (ideal body weight), monitoring again for adequacy of expiratory time. Throughout the course, efforts should be made to limit the alveolar plateau pressure (pressure at end-inspiration) to 25 cm H2O or less.
Fluid management is an important element of the care of patients with ARDS. Given the increased pulmonary capillary permeability in ARDS, further pulmonary edema accumulation is likely with any elevation in pulmonary hydrostatic pressures. Evidence in adults has shown that a “conservative” fluid strategy targeting lower cardiac filling pressures (CVP < 4 mm Hg, or, if a pulmonary artery catheter is used, pulmonary artery occlusion pressure < 8 mm Hg) is associated with better oxygenation and a shorter duration of mechanical ventilation compared to a “liberal” fluid strategy targeting CVP 10–14 mm Hg (or PAOP 14–18 mm Hg). Fluid restriction should only be implemented after hemodynamic variables stabilize and volume resuscitation should not be denied to hemodynamically unstable patients with ARDS.
Hemodynamic support is directed toward increasing perfusion and oxygen delivery. Patients should be given adequate intravascular volume resuscitation using either crystalloid or colloid solutions to restore adequate circulating volume, and inotropes or vasopressors should be titrated to achieve adequate end-organ perfusion and oxygen delivery. While blood transfusions are excellent volume expanders and should theoretically increase oxygen-carrying capacity, transfusion incurs the risks of volume overload and transfusion-related lung injury. There is no evidence to support transfusion above a normal hemoglobin level in ARDS patients.
Patients with ARDS require careful monitoring. Given the risks of ventilator-induced lung injury and the inherent limitations of pulse oximetry and capnography, arterial blood gas analysis is strongly preferred for accurate assessment of oxygenation and ventilation and careful titration of mechanical ventilation. Indwelling arterial catheters are useful for continuous blood pressure monitoring and frequent laboratory sampling. Many clinicians advocate the use of CVP measurements to help determine the level of cardiac preload, although it is important to emphasize that the CVP value must be interpreted in the context of intrathoracic pressure and myocardial compliance. For patients with severe disease or concurrent cardiac dysfunction, consideration can be given to pulmonary artery catheterization in order to guide fluid management and to allow assessment of mixed venous oxygen saturation as an index of overall tissue oxygenation. Since secondary infections are common and contribute to increased mortality rates, surveillance for infection is important by obtaining appropriate cultures and following the temperature curve and white blood cell count. Renal, hepatic, and GI function should be watched closely because of the prognostic implications of multiorgan dysfunction in ARDS.
For patients failing these standard approaches of mechanical ventilation and fluid restriction, several alternative or rescue therapies are available. High-frequency oscillatory ventilation (HFOV) has been used successfully for many years in pediatric patients with ARDS. No studies to date have compared HFOV to a modern lung protective conventional ventilation strategy, and whether HFOV provides any advantage over conventional ventilation for pediatric ARDS remains unknown. Earlier studies have demonstrated that pediatric ARDS patients treated with HFOV can demonstrate rapid and sustained improvements in oxygenation without adverse effects on ventilation, and have suggested that HFOV patients showed a reduced incidence of chronic lung injury, as evidenced by a decreased need for supplemental oxygen at 30 days. At present, whether HFOV is best used as a first-line ventilator strategy or as a rescue therapy for patients failing conventional ventilation remains a matter of institutional and clinician preference. Prone positioning is a technique of changing the patient’s position in bed from supine to prone, with the goal of improving ventilation of collapsed dependent lung units via postural drainage and improved ventilation-perfusion matching. This technique can dramatically improve gas exchange in the short term, particularly for patients early in the course of ARDS, but the gains are often not sustained. To date, clinical trials examining the role of prone positioning in both adults and children with ARDS have not shown any improvement in mortality or in duration of mechanical ventilation. Based on the ability of inhaled nitric oxide (iNO) to reduce pulmonary artery pressure and to improve the matching of ventilation with perfusion without producing systemic vasodilation, iNO can be used as a therapy for refractory ARDS. Several multicenter trials of iNO in the treatment of ARDS, both in adults and in children, showed acute improvements in oxygenation in subsets of patients, but no significant improvement in overall survival. As a result, iNO cannot be recommended as a standard therapy for ARDS. Surfactant-replacement therapy is also not routinely recommended for children with ARDS, as the data regarding its efficacy remain mixed. To date, it has been difficult to draw meaningful conclusions from the completed surfactant trials because they differ so greatly with respect to surfactant composition, dosing regimen, study population, and mechanical ventilation strategy. Finally, ECMO has been used to support pediatric patients with severe ARDS. Recent registry data suggest the overall survival rate for children who require ECMO for ARDS is around 40%–50%. To date, the efficacy of ECMO has not been evaluated against lung protective ventilation strategies for pediatric ARDS in a prospective randomized trial. In addition, recent improvements in outcome for pediatric ARDS patients receiving “conventional” therapies have made the role of ECMO less clear and have made further prospective randomized studies of ECMO difficult to complete. For now, ECMO remains a viable rescue therapy for patients with severe ARDS that is unresponsive to other modalities.
Outcomes
Information regarding the long-term outcome of pediatric patients with ARDS remains limited. One report of 10 children followed 1–4 years after severe ARDS showed that three children were still symptomatic and seven had hypoxemia at rest. Until further information is available, all patients with a history of ARDS need close follow-up of pulmonary function.
ARDS Definition Task Force: Acute respiratory distress syndrome: the Berlin definition. JAMA 2012;307(23):2526–2533 [PMID: 22797452].
Curley MA, Hibberd PL, Fineman LD et al: Effect of prone positioning on clinical outcomes in children with acute lung injury: a randomized controlled trial. JAMA 2005;294:229–237 [PMID: 16014597].
Duffett M et al: Surfactant therapy for acute respiratory failure in children: a systematic review and meta-analysis. Crit Care 2007;11:R66 [PMID: 17573963].
Randolph AG: Management of acute lung injury and acute respiratory distress syndrome in children. Crit Care Med 2009;37:2448–2454 [PMID: 19531940].
STATUS ASTHMATICUS
Pathogenesis
Life-threatening asthma exacerbations are caused by severe bronchospasm, excessive mucus secretion, inflammation, and edema of the airways (see Chapter 38). Reversal of these mechanisms is key to successful treatment. Several structural and mechanical features of the lungs of infants and children place them at increased risk for respiratory failure from severe asthma exacerbations, including less elastic recoil than the adult lung, thicker airway walls which lead to greater peripheral airway resistance for any degree of bronchoconstriction, increased airway reactivity to bronchoconstrictors, fewer collateral channels of ventilation, and a more compliant chest wall which can lead to increased work of breathing with airway obstruction. In addition, some individual patients display a pattern of recurrent life-threatening asthma exacerbations. These patients will often have a history of previous ICU admissions or intubations; obesity, lower socioeconomic status, and non-Caucasian race are additional risk factors for severe asthma exacerbations.
Clinical Findings
Patients presenting in status asthmaticus are often tachypneic and may have trouble speaking. Dyspnea at rest that interferes with the ability to speak can be an ominous sign of severe airflow obstruction. Accessory muscle use correlates well with expiratory flow rates less than 50% of normal predicted values. Inspiratory and expiratory wheezing, paradoxical breathing, cyanosis, and a respiratory rate more than 60 breaths/min are all important signs of serious distress. Particular attention should be paid to the degree of aeration. Diffuse wheezing is typically appreciated, but if severe airway obstruction reduces airflow enough, wheezing may be absent. Pulse oximetry should be performed on presentation. Saturations less than 90% on room air can be indicative of severe airway obstruction, especially in infants.
Patients with severe asthma exacerbations may display signs of panic or exhaustion and alterations in level of consciousness. Agitation, drowsiness, and confusion can be signs of elevated Paco2 levels and may signify impending respiratory failure. Likewise, gasping respirations or frank apnea are indications of respiratory failure and need for intubation.
Patients are typically tachycardic secondary to stress, dehydration, and β-agonist therapy. A pulsus paradoxus of over 22 mm Hg correlates with elevated Paco2 levels. Diastolic blood pressure may be low secondary to dehydration and β-agonist use. Diastolic pressures less than 40 mm Hg in conjunction with extreme tachycardia may impair coronary artery filling and predispose to cardiac ischemia, especially in teenagers.
Laboratory Findings
Blood gas measurements should be performed on all patients with severe asthma exacerbations. Venous blood gas measurements may serve as a screening test for acidosis and hypercapnia but cannot substitute for arterial blood gas measurements in critically ill asthmatics. Patients with severe asthma exacerbations typically have increased minute ventilation and should be expected to have a Paco2 less than 40 mm Hg. Normal to elevated Paco2 levels suggest respiratory failure. Metabolic acidosis may be due to relative dehydration, inadequate cardiac output, or underlying infection. Hypoxemia (Pao2 < 60 mm Hg) on room air may be a sign of impending respiratory failure or significant ventilation/perfusion mismatch caused by pneumonia or atelectasis. Ventilation/perfusion mismatching also can be exacerbated by β-agonist therapy due to effects on both the airway and vascular smooth muscle.
Monitoring of serum electrolytes may reveal decreased serum potassium, magnesium, and/or phosphate, especially in patients with prolonged β-agonist use. Blood count measurements are not required routinely. Leukocytosis is common in asthma exacerbations, and corticosteroid treatment causes demargination of polymorphonuclear leukocytes within a few hours of administration. Differentiating infection from stress demargination as causes of leukocytosis can be difficult; measurement of other inflammatory markers such as C-reactive protein (CRP) levels can be useful.
Measurement of forced expiratory volume in 1 second (FEV1) or peak expiratory flow (PEF) is recommended in the urgent or emergency care setting; however, patients with life-threatening asthma exacerbation are often unable to cooperate with testing. Values of less than 40% of predicted indicate a severe exacerbation and values less than 25% of predicted indicate imminent respiratory arrest. Repeated measures of pulmonary function in very severe exacerbations are of limited value. Electrocardiograms are not routinely recommended but may be indicated to rule out cardiac ischemia, especially in patients with known cardiac disease, extreme tachycardia and low diastolic blood pressure, or complaints of chest pain.
Chest x-rays should be obtained in severe asthma exacerbations to evaluate for treatable triggers such as pneumonia, foreign body aspiration, suspected air leak, or a chest mass. Particularly in patients with severe wheezing who lack a previous asthma history, alternative diagnoses such as foreign body aspiration, congestive heart failure, pulmonary infections, or mediastinal mass should be entertained. An expiratory chest film is particularly helpful in identifying foreign bodies. Pneumothorax and pneumomediastinum are common complications of severe asthma exacerbations and may occur in nonintubated patients.
Treatment
Much of the morbidity associated with the treatment of severe asthma is related to the complications of providing mechanical ventilation in patients with severe airflow obstruction. As a result, the goal of initial treatment of patients with life-threatening status asthmaticus is to improve their ability to ventilate without resorting to endotracheal intubation and mechanical ventilation. The medical therapies described in the following discussion should be undertaken swiftly and aggressively with the goal of reversing the bronchospasm before respiratory failure necessitates invasive ventilation.
Close monitoring of gas exchange, cardiovascular status, and mental status are crucial to assessing response to therapy and determining the appropriate interventions. Children with status asthmaticus require IV access, continuous pulse oximetry and cardiorespiratory monitoring. Due to inadequate minute ventilation and V/Q mismatching, patients with severe asthma are almost always hypoxemic and should receive supplemental humidified oxygen immediately to maintain saturations more than 90%.
The repetitive or continuous administration of a selective short-acting β2-agonist is the most effective means of rapidly reversing airflow obstruction. Treatment with agents such as albuterol remains the first-line therapy for these patients. If the patient is in severe distress and has poor inspiratory flow rates, thus preventing adequate delivery of nebulized medication, subcutaneous injection of epinephrine or terbutaline may be considered. The frequency of β2-agonist administration varies according to the severity of the patient’s symptoms and the occurrence of adverse side effects. Nebulized albuterol may be given intermittently at a dose of 0.1 mg/kg per nebulization up to 5.0 mg every 10–15 minutes, or it can be administered continuously at a dose of 0.5 mg/kg/h to a maximum of 20–30 mg/h, usually without serious side effects. IV β-agonists should be considered in patients with severe bronchospasm unresponsive to inhaled bronchodilators. The agent most commonly used in the United States is terbutaline, a relatively specific β2-agonist, which can be given as a bolus dose or as a continuous infusion. Owing to its relative specificity for β2-receptors, terbutaline has fewer cardiac side effects than previously available IV β-agonists such as isoproterenol. Terbutaline is given as a bolus or loading dose of 10 mcg/kg followed by a continuous infusion of 0.5–5 mcg/kg/min. The heart rate and blood pressure should be monitored closely, because excessive tachycardia, ventricular ectopy, and diastolic hypotension may occur in patients receiving either inhaled or IV β2-agonist therapy. In general, patients receiving IV therapy should have indwelling arterial lines for continuous blood pressure and blood gas monitoring.
Immediate administration of systemic corticosteroids is critical to the early management of life-threatening status asthmaticus. Although oral systemic corticosteroids are generally recommended, consideration should be given to IV steroid administration in critically ill patients secondary to frequent intolerance of enterally administered medications. A dose of 2 mg/kg/d of methylprednisolone is generally prescribed for the critical care setting.
Infants and children with status asthmaticus may become dehydrated as a result of increased respiratory rate and decreased oral intake. In these patients, clinicians should make an assessment of fluid status and provide appropriate corrections. Fluid replacement should be aimed toward restoration of euvolemia while avoiding overhydration. The hemodynamic effects of high dose β2-agonist therapy (peripheral dilation, diastolic hypotension) may require some fluid resuscitation to maintain cardiac output and avoid metabolic acidosis. Antibiotics are generally not recommended for treatment of status asthmaticus unless a coexisting infection is identified or suspected.
For severe exacerbations unresponsive to the initial treatments listed above, additional treatments may be considered to avoid intubation. Ipratropium bromide, an inhaled anticholinergic bronchodilator, is a reasonable intervention given its low side-effect profile, though two controlled clinical trials failed to detect a significant benefit from its addition to standard therapy in preventing hospitalization due to asthma. Magnesium sulfate is reported to be an effective bronchodilator in adult patients with severe status asthmaticus when given in conjunction with steroids and β2-agonists and may be considered for patients with impending respiratory failure or who have life-threatening exacerbations that do not respond well to the first 1 hour of intensive conventional therapy. The mechanism of action of magnesium is unclear, but its smooth muscle relaxation properties are probably caused by interference with calcium flux in the bronchial smooth muscle cell. Magnesium sulfate is given IV at a dose of 25–50 mg/kg per dose. Although usually well tolerated, hypotension and flushing can be side effects of IV magnesium administration. Heliox-driven albuterol nebulization can also be considered for patients refractory to conventional therapy. Heliox is a mixture of helium and oxygen that is less viscous than ambient air and can improve airway delivery of albuterol and gas exchange. Clear evidence of clinical efficacy is lacking for young patients, and heliox requires a mixture containing at least 60%–70% helium to alter viscosity sufficiently to significantly improve air flow, limiting its use in patients requiring higher concentrations of supplemental oxygen.
Theophylline is a methylxanthine that remains controversial in the management of severe asthma. Clinical studies have yielded a mixed verdict on its benefit when given with steroids and β2-agonists for children with asthma. This uncertainty, together with its high side-effect profile, led to a general recommendation against the use of theophylline for asthma exacerbations, although it may still have a role in severe exacerbations as a means to prevent intubation. The theoretical benefit of theophylline is relaxation of airway smooth muscle by preventing degradation of cyclic guanosine monophosphate, a mechanism of action distinct from that of β2-agonists. Besides causing bronchodilation, this agent decreases mucociliary inflammatory mediators and reduces microvascular permeability. However, the pharmacokinetics of theophylline are erratic and therapeutic levels can be difficult to manage. It is also associated with serious side effects, such as seizures and cardiac arrhythmias that can occur with high drug levels. Theophylline is given IV as aminophylline. Each 1 mg/kg of aminophylline given as a loading dose will increase the serum level by approximately 2 mg/dL. For a patient who has not previously received aminophylline or oral theophylline preparations, load with 7–8 mg/kg of aminophylline in an attempt to achieve a level of 10–15 mg/dL; then start a continuous infusion of aminophylline at a dosage of 0.8–1 mg/kg/h. A postbolus level and steady-state level should be drawn with the initiation of the medication. Watch closely for toxicity (gastric upset, tachycardia, and seizures) and continue to monitor steady-state serum levels closely, trying to maintain steady-state levels of 12–14 mg/dL.
Noninvasive ventilation (NIV) is another approach for treatment of respiratory failure due to severe asthma exacerbation that may help avoid the need for intubation and mechanical ventilation. Positive pressure ventilation may help to avoid airway collapse during exhalation as well as to unload fatigued respiratory muscles by reducing the force required to initiate each breath. Because of its noninvasive interface, spontaneous breathing and upper airway function are preserved, allowing the patient to provide his/her own airway clearance. Data on the effectiveness of NIV for acute severe asthma in children are limited to small studies and case series but have shown an improvement in gas exchange and respiratory effort.
If aggressive management fails to result in significant improvement, mechanical ventilation may be necessary. Patients who present with apnea or coma should be intubated immediately. Otherwise, if there is steady deterioration despite intensive therapy for asthma, intubation should occur semi-electively before acute respiratory arrest, because the procedure can be dangerous in patients with severe asthma given the high risk of barotrauma and cardiovascular collapse. Mechanical ventilation for patients with asthma is difficult because the severe airflow obstruction often leads to very high airway pressures, air trapping, and resultant barotrauma. The goal of mechanical ventilation for an intubated asthma patient is to maintain adequate oxygenation and ventilation with the least amount of barotrauma until other therapies become effective. Worsening hypercarbia following intubation is typical, and aggressive efforts to normalize blood gases may only lead to complications. Due to the severe airflow obstruction, these patients will require long inspiratory times to deliver a breath and long expiratory times to avoid air trapping. In general, the ventilator rate should be decreased until the expiratory time is long enough to allow emptying prior to the next machine breath. Ventilator rates of 8–12 breaths/min are typical initially. Either volume- or pressure-targeted modes of ventilation can be used effectively, although tidal volume and pressure limits should be closely monitored. As a patient moves toward extubation, a support mode of ventilation is useful, as the patient can set his or her own I-time and flow rate. Due to air trapping, patients can have significant auto-PEEP. The level of PEEP on the ventilator is usually set low (0–5 cm H2O) to minimize air trapping and high peak pressures. Isolated reports have noted patients who respond to greater PEEP, but these are exceptions.
These ventilator strategies and resulting hypercarbia typically are uncomfortable, requiring that patients be heavily sedated and often medically paralyzed. Fentanyl and midazolam are good choices for sedation. Ketamine is a dissociative anesthetic that can be used to facilitate intubation and also as a sedative infusion for intubated patients. Ketamine has bronchodilatory properties, although it also increases bronchial secretions. Barbiturates should be avoided as well as morphine, both of which can increase histamine release and worsen bronchospasm. Most patients, at least initially, will also require neuromuscular blockade to optimize ventilation and minimize airway pressures. In intubated patients not responding to the preceding strategies, inhaled anesthetics, such as isoflurane, should be considered. These agents act not only as anesthetics but also cause airway smooth muscle relaxation; they must be used with caution, however, as they can also cause significant hypotension due to vasodilation and myocardial depression.
Prognosis
Status asthmaticus remains among the most common reasons for admission to the PICU. It is associated with a surprisingly high mortality rate (1%–3%), especially in patients with a previous PICU admission. As many as 75% of patients admitted to the PICU with life-threatening asthma flares will be readmitted with a future exacerbation, emphasizing the need for careful outpatient follow-up of this high-risk population.
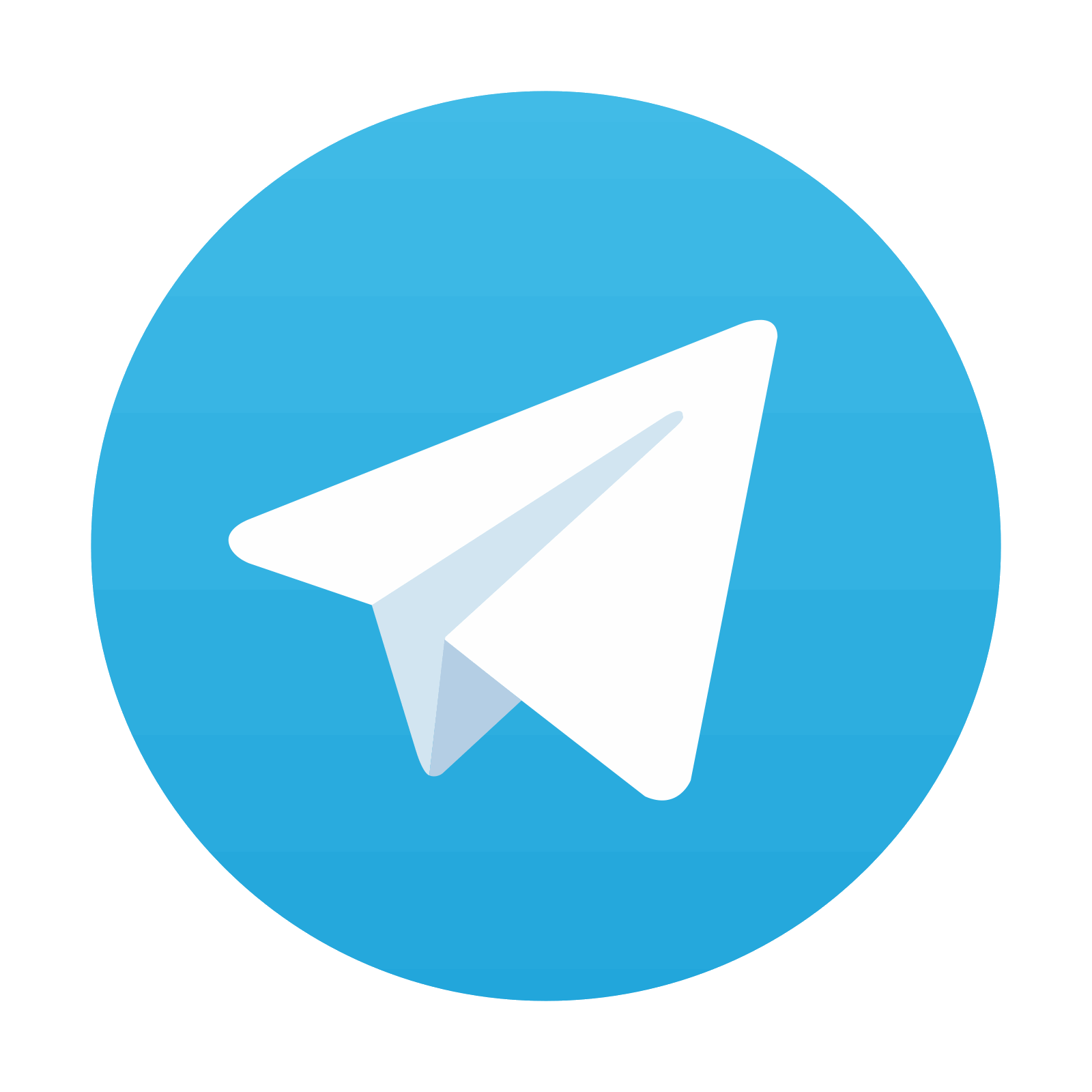
Stay updated, free articles. Join our Telegram channel
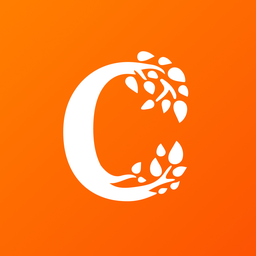
Full access? Get Clinical Tree
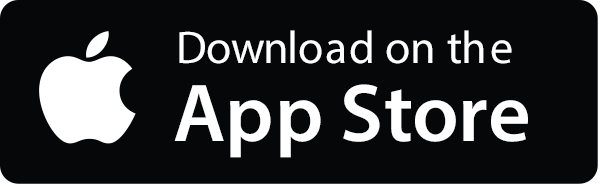
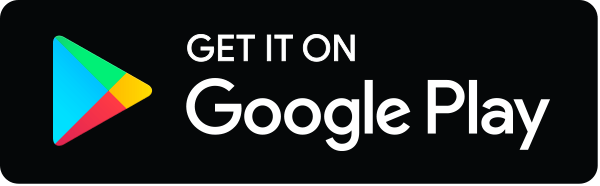