and David J. Durand9
(8)
Section of Neonatology, Department of Pediatrics, University of Arkansas for Medical Sciences, Arkansas Children’s Hospital, 1900 Maryland, Little Rock, AR 72202, USA
(9)
Department of Physiology and Pediatrics, Medicine, Temple University School of Medicine, Philadelphia, PA 19104, USA
Educational Aims
-
To be aware of the history of HFOV, both animal studies and clinical trials
-
To understand the major components of HFOV physiology
-
To describe clinical situations in which HFOV may be useful
-
To understand the basic ventilator management required during HFOV use
22.1.1.1 Introduction
High-frequency oscillatory ventilation (HFOV) is now a mainstay of respiratory care for the neonatal patient. In this chapter, we will define HFOV as those ventilators with a “true” active expiratory phase created by a piston or diaphragm. Jet ventilation and flow interrupters are discussed elsewhere in this book.
22.1.1.2 History of HFOV: The Animal Studies
In 1915, Henderson et al. observed that panting dogs were able to maintain adequate alveolar ventilation while breathing at high frequencies. They postulated that maintenance of normal ventilation with tidal volumes of less than the anatomic dead space might be occurring (Henderson et al. 1915). These findings were confirmed by Briscoe et al. in 1954 in the first description of high-frequency ventilation (Briscoe et al. 1954). Through the 1970s, a series of investigations demonstrated that HFOV could effectively support gas exchange (Jonzon et al. 1971, 1973; Sjostrand 1977; Sjostrand and Eriksson 1980).
In the 1980s, intense animal and human research on HFOV confirmed it as a viable technique for ventilation. The work of deLemos and colleagues using the infant baboon model set the stage for human trials and found that HFOV, when compared to the conventional ventilation used at that time, resulted in less lung injury (deLemos et al. 1987, 1989; Meredith et al. 1989; Kinsella et al. 1991). By the early 1990’s, there was a wealth of information from multiple investigators, using multiple animal models, suggesting that HFOV caused less injury to the immature lung than did “conventional ventilation” (Hamilton et al. 1983; Truog et al. 1984; McCulloch et al. 1988; Jackson et al. 1994). The generally accepted mechanism of this superiority was the ability to support gas exchange with small tidal volumes and high mean airway pressure, thereby minimizing injury from both overdistention and atelectasis.
22.1.1.3 History of HFOV: The Clinical Trials
Though the superiority of HFOV over conventional ventilation (CV) has not been proven in the human neonate with respiratory distress syndrome (RDS), its equivalence has been clearly demonstrated when compared to modern techniques of CV that focus on minimizing barotrauma and volutrauma.
The HiFi study of 1989 was the first large multicenter trial of HFOV compared to CV. At that time investigators and clinicians were still learning how to best apply this new technology. Though the HiFi trial showed no benefit of HFOV and possible increases in intraventricular hemorrhage (IVH) and periventricular leukomalacia (PVL), the use of an optimal mean airway pressure to open the lung was not applied in that study (HiFi Study Group 1989).
Multiple smaller studies of HFOV vs. CV followed the HiFi trial, some of which showed benefit of HFOV and some, equivalence. No study showed increased lung damage in patients receiving HFOV. Clinical trials on infants showed that HFOV could be safe and effective (Clark et al. 1992; HiFO Study Group. 1993; Ogawa et al. 1993; Gerstmann et al. 1996), and an oscillator was approved in the United States for clinical use in neonates in 1991, the SensorMedics 3100.
Two large randomized trials were published in 2002. Courtney et al. found, in 500 very low birth weight infants, a reduction in bronchopulmonary dysplasia (BPD) and time on ventilator support when infants who required surfactant were treated with HFOV within 6 h of birth and were extubated directly to nasal continuous positive airway pressure (nCPAP) (Courtney et al. 2002). Johnson et al. found no difference in BPD in over 800 infants randomized at birth and treated with HFOV for a minimum of 5 days (Johnson et al. 2002).
A Cochrane meta-analysis of the HFOV trials found no evidence for benefit or harm when HFOV was compared to CV in infants with RDS (Cools et al. 2009) A recent individual patient data meta-analysis confirmed these results (Cools et al. 2010). The predominant potential benefit assessed in these trials was reduction in death and/or chronic lung disease; the predominant adverse effects assessed were severe IVH and PVL.
An important caveat in interpreting these clinical trials and the meta-analyses is that the trials were conducted in an era when ventilator management of very low birth weight infants was different than current approaches. Many of the babies included in these studies would probably not be ventilated at all or would be extubated much earlier in the current era. In addition, our current understanding of the multifactorial nature of chronic lung disease makes the results of these studies less surprising (Baraldi and Filippone 2008). It is also reassuring that early concerns of possible increased neurological damage with HFOV have not been borne out.
22.1.1.4 Physiology of HFOV
Chang, in his classic article of 1984, described the mechanisms of gas transport during HFOV (Chang 1984). The physics of gas exchange with HFOV are complex and dealt with more fully elsewhere in this text. In brief, HFOV can be broadly described as enhanced mixing between gas in the upper airway and gas in the alveoli. While the mean airway pressure holds the lung open and promotes oxygenation, the oscillations essentially “shake” the gas, promoting rapid and efficient gas exchange. As HFOV amplitude is increased, there is increased shaking, which results in increased mixing and increased gas exchange.
An essential part of HFOV physiology is the role the upper airway plays as a low-pass filter between the upper airway and the alveoli, where high frequencies are attenuated or dampened more than low frequencies. For example, at 1 Hz (60 breaths/min), essentially all of the pressure seen in the upper airway with each ventilator inflation is transmitted to the alveoli. However, at 10 Hz (600 breaths/min), there is significant attenuation of the amplitude signal by the airways. At 20 Hz, the attenuation is even more pronounced, causing even less of the amplitude pressure to be transmitted to the airway. Thus, changes in HFOV frequency have profound effects on the amount of pressure transmitted to the alveoli and on the efficacy of gas mixing.
22.1.1.5 When to Consider HFOV
22.1.1.5.1 General Considerations
HFOV should be considered early in the course of respiratory failure, prior to lung damage from volutrauma and/or barotrauma. Clearly when CV cannot be employed safely – when high volumes and pressures must be used to effect gas exchange – HFOV and other forms of high-frequency ventilation can be lifesaving.
“Rescue” of the lungs following attempts to sustain oxygenation and ventilation with high tidal volumes or high pressures is seldom successful or at best may salvage an infant who already has sustained significant lung damage. Infants on CV with sufficient positive end expiratory pressure (PEEP) and who require tidal volumes of more than 6 ml/kg or peak inspiratory pressures of greater than 25 cm H2O should be considered for some form of high-frequency ventilation. HFOV may be very effective for the following conditions.
22.1.1.5.2 Respiratory Distress Syndrome
RDS can be treated extremely effectively with HFOV. First-intention use, upon arrival of the infant to the NICU, is preferred by some centers (Rimensberger et al. 2000; De Jaegere et al. 2006). Others use HFOV for infants who do not wean quickly from CV after surfactant is given or whose condition worsens. Requirement of dangerously high tidal volumes or peak inspiratory pressures necessitates intervention, usually with HFOV. Surfactant can be given during HFOV without disconnecting the ventilator by using an in-line catheter.
22.1.1.5.3 Persistent Pulmonary Hypertension (PPHN) and Meconium Aspiration Syndrome (MAS)
Though PPHN and MAS are not always coexistent, they often occur together. HFOV is the treatment of choice for PPHN with lung disease requiring use of inhaled nitric oxide (iNO). Kinsella et al. found that significantly more infants responded to HFOV plus iNO than with iNO and CV or with HFOV alone (Kinsella et al. 1997).
22.1.1.5.4 Abdominal Surgery/NEC
Infants who require abdominal surgery, such as with omphalocele or gastroschisis, or infants who develop NEC may require high ventilator settings due to abdominal distention. These infants may also have concurrent lung disease. Use of HFOV in these situations can support the infant during the recovery period.
22.1.1.5.5 Hypoplastic Lungs
Infants with hypoplastic lungs from a variety of causes such as Potter’s sequence or certain skeletal anomalies may require ventilator support for long periods of time. Use of CV in these babies may require unsafe parameters and may often lead to air leak. HFOV can provide long-term support for potentially viable infants with lung hypoplasia.
22.1.1.5.6 Surgery
Nearly any surgical procedure can be performed while an infant is on HFOV. A pediatric anesthesiologist or neonatologist skilled in its management should be at the bedside. Periodic assessment of blood gases or use of both continuous saturation and transcutaneous CO2 monitoring should be done. Patent ductus arteriosus (PDA) ligation on HFOV is done at the bedside during HFOV in many NICU’s. Stability of the lung inflation during lung retraction in PDA ligation is often better than with CV, and the infant may therefore better tolerate the surgery. Other surgical procedures, such as laparotomy for NEC, are also often performed during HFOV.
22.1.1.5.7 Congenital Diaphragmatic Hernia (CDH) and Other Conditions
HFOV is often needed during treatment of CDH to prevent the need for high peak pressures during CV and to prevent air leak. Many other, more rare, congenital anomalies may also respond to use of HFOV. Hydrops fetalis, chylothorax, and cystic adenomatoid malformation are some examples.
22.1.1.5.8 Extracorporeal Membrane Oxygenation (ECMO)
Infants on ECMO can be managed on HFOV. Care must be taken to assure that the vibrations of the oscillator do not dislodge the ECMO cannulas.
22.1.1.6 Management of the Infant on HFOV
The lung, especially when becoming diseased or recuperating from disease, is a dynamic organ with changes in its mechanical properties that will depend on the disease process, the stage of that process, and the interventions we impose. We must be diligent about not only how and when we increase ventilator settings but how and when we wean them so that we do not create iatrogenic complications and lung damage.
22.1.1.6.1 Mean Airway Pressure (MAP)
As with any form of ventilation, it is critically important to recruit the lung. Lung recruitment during HFOV is best done by increasing the mean airway pressure (MAP) until the FiO2 is at a minimum. At that point, the MAP can be slowly decreased until the FiO2 starts to rise. The MAP is then set at the point just before this rise in FiO2 occurred. The infant is thus ventilated on the descending limb of the pressure-volume curve (Tingay et al. 2006).
22.1.1.6.2 Frequency and Amplitude
In HFOV, frequency is typically measured in Hertz (Hz), where 1 Hz = 1 cycle/s or 60 cycles/min. Optimal frequency depends on the size of the patient and the underlying lung disease. Most oscillators function between 3 and 20 Hz. However, in the neonate, 8–12 Hz appears to be the most effective. Higher frequency may result in air trapping due to the shortened expiratory time. Lower frequency results in large increases in tidal volume. For most preterm infants with restrictive lung disease such as RDS, we recommend 10–12 Hz. Term infants with obstructive disease such as meconium aspiration syndrome may require 8 Hz.
Amplitude should be that which maintains the PCO2 at the desired level. We recommend transcutaneous CO2 monitoring for infants on HFOV. Oscillators are powerful ventilators and PCO2 can be easily driven to dangerously low levels very quickly. Low PCO2 in the preterm infant has been associated with PVL (Shankaran et al. 2006).
22.1.1.6.3 Inspiratory Time
The recommendations of the manufacturer should be followed. Some oscillators, such as the Humming series, have a fixed inspiratory time (IT) of 50 % of the respiratory cycle. Others, such as the Babylog 8000, vary with the frequency. Others, such as the SensorMedics 3100A, may be operator-set; however, the manufacturer recommends a 33 % IT, due to lack of data as to the effects of a longer percent inspiratory phase.
22.1.1.6.4 Flow
Increased flow can cause increased turbulence in the airways and, therefore, increased resistance. The lowest flow at which the MAP is maintained is appropriate.
22.1.1.6.5 Weaning
As previously mentioned, timely and appropriate weaning is crucial to prevent complications and lung damage. Particularly following surfactant administration, the compliance of the lung may improve rapidly, necessitating rapid weaning as well. With proper lung recruitment, the FiO2 required by most infants will be low, nearly always below 0.40 and often below 0.30. Once the infant is stable at this point with acceptable blood gases and a chest radiograph that shows good lung recruitment, MAP can be weaned. Rate of wean will depend upon the underlying lung disease. An infant with RDS who has responded to surfactant can often be weaned from HFOV within hours.
Changes in MAP require the lung to recruit or derecruit, a process that takes a little time, depending on the degree of parenchymal disease. MAP changes should usually be made no more frequently than every 30–60 min to allow for stabilization of the lung at the new MAP. Any evidence of overdistention, such as flat diaphragms or small heart on chest radiograph, should be accompanied by aggressive weaning of MAP. Amplitude can be weaned as needed to keep the PCO2 in an acceptable range.
22.1.1.6.6 Care of the Infant on HFOV
Infants on HFOV do not require increased sedation. They are usually quite comfortable and may even become apneic. Unlabored breathing is perfectly normal during HFOV; labored breathing should alert the caregiver to the possible need for increased ventilator support. Sedation and pain relief should be used as needed for procedures and discomfort.
We recommend in-line suctioning for infants on HFOV to maintain the lung volume and assure accurate positioning of the suction catheter tip, as well as for infection control.
Infants on HFOV can be positioned prone or supine. They can be nursed under a radiant warmer or in an incubator. They can be held by the parents. Infants should be repositioned at least every 12 h to prevent pressure sores. Repositioning the infant can be done without disconnecting the ventilator.
22.1.1.6.7 Extubation
Infants can be extubated directly from HFOV to nCPAP. In general, amplitude should be weaned so that the infant is breathing spontaneously above the ventilator prior to extubation. Extubation parameters include MAP of 6–8 cm H2O, relatively clear chest radiograph, and FiO2 of 0.30 or less in most cases. Small babies will benefit from a caffeine load prior to extubation. Some infants may be successfully extubated from HFOV at even higher levels of MAP and FiO2.
22.1.1.7 Conclusion
HFOV has increased our options for the care of many newborn infants with significant respiratory problems. After more than three decades of research and clinical use, it is clear that (1) HFOV is an effective technique for gas exchange; (2) HFOV is at least as safe as conventional ventilation; and (3) if there are advantages to HFOV over conventional ventilation, it is for cases where it is used to prevent lung injury caused by overdistention and/or atelectasis, particularly in severe restrictive disease. It must be used properly, as with any mechanical ventilator, to assure the best response and to avoid preventable complications.
Essentials to Remember
-
HFOV is at least equivalent to conventional ventilation in infants with RDS and may provide significant advantages in some circumstances and other disease states.
-
HFOV is not associated with adverse effects either in the lung or in the brain.
-
As with any form of ventilation, it is critically important to appropriately recruit the lung during HFOV.
-
Infants may be successfully extubated directly from HFOV to nCPAP.
22.1.2 Pediatric HFOV
Gerhard K. Wolf10, 12 and John H. Arnold10, 11
(10)
Division of Critical Care Medicine, Department of Anesthesiology, Perioperative and Pain Medicine, Children’s Hospital Boston, Harvard Medical School, 300 Longwood Avenue, Boston, MA 02115, USA
(11)
Children’s Hospital MSICU Office, Farley 517, 300 Longwood Avenue, Boston, MA 02115, USA
(12)
Associate in Critical Care Medicine, Harvard Medical School, Boston, USA
Educational Aims
-
Reviewing the evidence-based use of HFOV in pediatric hypoxemic respiratory failure
-
Assessing the patients that are considered for a trial of HFOV
-
Initiation of HFOV in pediatric patients
-
Reviewing the patient population that may not benefit from HFOV
22.1.2.1 Evidence for High-Frequency Ventilation in Pediatric Patients
High-frequency oscillatory ventilation (HFOV) has been compared to conventional ventilation in neonatal (Courtney et al. 2002, Johnson et al. 2002), pediatric (Arnold et al. 1994), and adult (Derdak et al. 2002) trials. Although none of the studies showed an improvement in mortality or ventilator-free days during HFOV, there is a large body of evidence that HFOV is a safe strategy of ventilation allowing rapid and effective recruitment of lung volume, without significantly increased adverse events during HFOV as compared to conventional ventilation.
Data from a neonatal trial (Courtney et al. 2002) indicated a small benefit of HFOV in terms of pulmonary outcome for very low birth weight infants. A trial in pediatric patients (Arnold et al. 1994) showed a significant improvement in oxygenation during HFOV compared with a conventional ventilatory strategy and a decreased need for supplemental oxygen at 30 days. Aggressive recruitment of lung volume during HFOV is often achieved using higher mean airway pressures compared to conventional ventilation. A multicenter experience from different pediatric intensive care units in the United States demonstrated significant increases in mean airway pressure and concomitant increases in oxygenation index when patients were transitioned from conventional ventilation to HFOV (Arnold et al. 2000). HFOV has been advocated as a rescue strategy in patients who are failing conventional ventilation, but smaller single-center studies in children (Fedora et al. 2000; Ben Jaballah et al. 2005) and adults (Mehta et al. 2001) also suggested some benefit towards an early implementation of HFOV. However, an early use of HFOV often implies administering neuromuscular blocking agents to a patient early in the course of disease, which may have to be weighed carefully against potential side effects of prolonged neuromuscular blockade.
Utilizing HFOV as a recruitment strategy may result in increased delivery of inhaled gases such as inhaled nitric oxide (iNO) to recruited lung areas. Utilizing data from a randomized, controlled multicenter trial of the use of iNO in pediatric acute hypoxemic respiratory failure, one study indicated that the use of HFOV plus iNO resulted in a greater improvement in oxygenation compared to a strategy combining conventional ventilation and iNO (Dobyns et al. 2002).
A randomized multicenter trial comparing HFOV to conventional ventilation in adult patients with acute respiratory distress syndrome (Derdak et al. 2002) resulted in an improvement in early oxygenation during HFOV using an aggressive lung recruitment strategy; however, this effect was lost after 24 h. The early positive oxygenation response during HFOV may be explained by the prevalence of higher mean airway pressures during HFOV as compared to conventional ventilation. There were no significant differences in mortality or ventilator-free days between the groups. The use of HFOV in this study was not associated with increased adverse hemodynamic effects, evidence of barotrauma, or mucous plugging when compared to conventional ventilation.
22.1.2.2 Ventilators Used for High-Frequency Ventilation in the Pediatric Population
There are a variety of high-frequency devices available. Most devices are reserved for neonatal patients and small infants. The SensorMedics 3100 A/B (Viasys, Yorba Linda, CA) is the only device that generates effective gas exchange in neonates, children, and adults. Two devices are available. The SensorMedics 3100 A is being used for neonates and children, and the SensorMedics 3100 B is approved for adults and larger children over 35 kg. For the 3100 A, the bias flow ranges from 0 to 40 l/min, mean airway pressures range from 3 to 45 cm H2O, and the frequency ranges from 3 to 15 Hz (180–900 breaths/min). In comparison to the 3100 A, the 3100 B has a more powerful diaphragm, can provide a larger bias flow (0–60 l/min), and can apply higher mean airway pressures of up to 55 cm H2O.
22.1.2.3 Initiating HFOV in Pediatric Respiratory Failure
22.1.2.3.1 Indication and General Considerations
HFOV is considered when conventional modes of ventilation fail to provide adequate oxygenation or adequate alveolar ventilation in pediatric patients with acute respiratory distress syndrome (ARDS). Failing conventional ventilation can be indicated by arterial hypoxemia despite a FiO2 ≥0.7 and a mean airway pressure exceeding 15 cm H2O. Recent pediatric multicenter trials involving ventilation algorithms have used the oxygenation index (OI) (FiO2 • mean airway pressure • 100/PaO2) to determine the transition from conventional ventilation to HFOV; the transition to HFOV was considered when the OI was 15 and rising and the use of HFOV was mandated with an OI of 20 (Curley et al. 2005; Fineman et al. 2006).
Patients transitioning to HFOV should have an arterial line for invasive blood pressure and arterial blood gas monitoring and central venous access for monitoring of the central venous pressure. While neonates can breathe spontaneously during HFOV, the bias flow of the system is not sufficiently high to support spontaneous ventilation in pediatric patients (van Heerde et al. 2006). This effect was demonstrated in a study using an artificial lung device during HFOV. The work of breathing was considerably increased when an (simulated) adult or larger pediatric patient was breathing spontaneously during HFOV. During the simulation of a newborn breathing spontaneously, the work of breathing was markedly reduced (van Heerde et al. 2006). Outside the neonatal population, neuromuscular paralysis is required in patients to prevent depressurization of the circuit resulting in alveolar derecruitment (Heulitt et al. 2008; Wolf and Arnold 2007).
22.1.2.3.2 Considerations for Exclusion
22.1.2.3.2.1 Obstructive Airway Disease
Diseases with increased airway resistance as seen in status asthmaticus, bronchiolitis, and reactive airway disease are generally associated with hypercarbia and air trapping rather than arterial hypoxemia. The application of an aggressive recruitment strategy may increase the incidence of air trapping and the risk of extrapulmonary leak. However, infants with respiratory syncytial virus (RSV) infections may present with both acute hypoxemic respiratory failure due to acute lung injury and impaired CO2 elimination secondary to small airway obstruction. The successful use of HFOV in infants with RSV infection has been reported in a few cases, leading to reversal of hypoxemia and recruitment of atelectasis while achieving adequate CO2 removal (Berner et al. 2008). The use of HFOV in intubated children with status asthmaticus to facilitate CO2 removal has also been reported (Duval and van Vught 2000). However, utilizing HFOV to achieve adequate CO2 removal in this setting often requires the combination of a high amplitude and a low device frequency, resulting in increased delivered tidal volumes during HFOV. Since delivered tidal volumes are not measured during HFOV and may be as high as 5 ml/kg during clinical use (Sturtz et al. 2008), a high-amplitude low-frequency strategy may result in increased lung overdistention and may compromise the lung protective effects of HFOV (Wolf and Arnold 2008).
22.1.2.3.2.2 Hemodynamic Considerations
Patients with uncorrected hypotension should be adequately volume-resuscitated and stabilized on vasopressors before the initiation of HFOV. Cardiac conditions with passive pulmonary blood flow dependency such as a Fontan circulation are a relative contraindication to HFOV, as the right ventricular preload may be further impeded with escalating mean airway pressures.
Essential to Remember
-
There is a large body of evidence that HFOV is a safe strategy of ventilation allowing rapid and effective recruitment of lung volume, without significantly increased adverse events during HFOV as compared to conventional ventilation.
-
HFOV is considered when conventional modes of ventilation fail to provide adequate oxygenation or adequate alveolar ventilation in pediatric patients with acute respiratory distress syndrome.
-
Patients with uncorrected hypotension should be adequately volume-resuscitated and stabilized on vasopressors before the initiation of HFOV.
-
Diseases with increased airway resistance (status asthmaticus, bronchiolitis, and reactive airway disease) that are associated with hypercarbia and air trapping rather than arterial hypoxemia may be relative contraindications to HFOV.
22.2 Clinical Use of High-Frequency Jet Ventilation (HFJV)
Martin Keszler13
(13)
Department of Physiology and Pediatrics, Medicine, Temple University School of Medicine, Philadelphia, PA 19104, USA
Educational Aims
-
Describe historical background and current spectrum of clinical use of HFJV
-
Summarize the determinants of gas exchange with HFJV
-
Describe specific pathophysiologies and the principles that guide clinical use of HFJV in each condition
Initial use of HFJV in the 1980s focused on treatment of air leak, and to that end a ventilation strategy evolved that emphasized the use of minimal peak and mean airway pressures in order to facilitate resolution of the trapped air (Spitzer et al. 1989). As emphasis shifted from late rescue therapy to earlier application and as laboratory and clinical evidence regarding the importance of the open lung concept emerged, it became apparent to a growing number of clinicians that a modification of that approach was needed (Keszler et al. 1997). Over time, we learned the importance of tailoring ventilation strategy to the underlying disease pathophysiology and the value of individualized patient care. Although different authors have divided pulmonary disorders in a variety of ways, it is useful to think of the key element in the various underlying disorder in six categories described in Table 22.1: uniform atelectatic lung disease, severe nonuniform disease, air leak syndromes, obstructive lung disease, lung hypoplasia, and restrictive lung disease.
Table 22.1
Classification of neonatal pulmonary disorders based on underlying pathophysiology
Description |
Example |
Key pathophysiologic feature |
---|---|---|
Uniform atelectatic disease |
RDS, ARDS, diffuse pneumonia, surfactant inactivation from pulmonary hemorrhage, meconium aspiration |
Short time constants, relatively uniform, prone to atelectasis, easily recruitable |
Air leak syndromes |
PIE, pneumothorax, bronchopleural fistula, tracheoesophageal fistula |
Usually coexists with atelectatic or nonuniform disease – thus conflicting imperatives to minimize leak but avoid atelectasis. PIE adds elements of restriction and obstruction |
Nonuniform disease |
MAS, other aspiration syndromes, patchy atelectasis, lobar pneumonia, some patients with BPD |
Highly variable regional compliance and resistance, prone to air trapping and air leak, may be complicated by pulmonary hypertension. Early stages of MAS add element of obstruction. Pathophysiology changes over time and can be highly variable |
Obstructive disease |
Early MAS, BPD |
Increased airway resistance is the key element, typically combined with nonuniform disease and tendency for airway collapse. Prolonged time constants and air trapping are prominent features |
Lung hypoplasia |
CDH, PPROM, oligohydramnios, renal agenesis |
Small, atelectasis-prone lungs, highly susceptible to volutrauma and air leak. Unrepaired diaphragmatic hernia adds element of restrictive disease. Pulmonary hypertension commonly present |
Restrictive disease |
Severe abdominal distention, severe chest wall edema, severe diffuse PIE, pleural effusion, unrepaired CDH |
Lung expansion and excursion are limited by intrapulmonary or extrapulmonary space occupying lesion or external chest wall restriction. Hemodynamic compromise is common. May occur in combination with a variety of other pathophysiologies |
22.2.1 Basic Principles of Controlling Gas Exchange with HFJV
The ventilator settings on the Bunnell Life Pulse ventilator are analogous to those of conventional ventilation, except that PEEP is controlled by the tandem conventional ventilator which also provides the option of superimposing background sigh/recruiting inflations (Harris and Bunnell 1993).
22.2.1.1 Ventilator Rate
Ventilator rate is set and displayed in “breaths”/min with a default setting of 420/min = 7 Hz, a value found in early studies to be optimal for a typical preterm infant with RDS. The ventilator rate has only a minor impact on CO2 exchange (unless it is too high and is causing air trapping) and is normally adjusted infrequently. Like conventional ventilation, HFJV depends on passive exhalation and adequate expiratory time is necessary to avoid air trapping. The optimal rate is a function of time constants. Faster rate is appropriate and safe with uniform atelectatic disease, especially in small infants. Larger infants have longer time constants and need slower rates. Infants with obstructive lung disease (MAS, BPD) also have longer time constants. Typical rate is 420–500 for very small infants with RDS and as low as 280–320 with large infants with MAS. As RDS evolves into BPD with resulting longer time constants, ventilator rate may need to be lowered to avoid air trapping.
22.2.1.2 Inspiratory Time
The default value of inspiratory time is 0.02 s and remains unchanged under most clinical condition. The shortest possible inspiratory time allows maximum expiratory time to minimize the risk of air trapping. Only in larger infants beyond the neonatal period who are ventilated with respiratory rate ≤300 is a small increase in inspiratory time sometimes desirable in order to achieve larger tidal volume at maximum PIP.
22.2.1.3 Peak Inspiratory Pressure
PIP is adjusted primarily to control ventilation with a lesser effect on oxygenation. Pressure amplitude (PIP-PEEP) directly determines tidal volume for any given lung compliance. Increasing PIP with constant PEEP will improve ventilation. It is critical to recognize that improving lung compliance will result in larger tidal volume at any given pressure amplitude. Because of the geometric relationship between V T and CO2 removal, even small changes in V T can result in substantial change in ventilation. When compliance improves after lung volume recruitment or surfactant administration or for any other reason, inadvertent hyperventilation may occur rapidly – for this reason, the use of transcutaneous CO2 monitoring is strongly encouraged. PIP has a less dramatic effect on oxygenation, because the inspiratory/expiratory ratio is very short. This means that the contribution of PIP to mean airway pressure is very small. However, substantial lowering PIP without increasing PEEP to maintain mean airway pressure may result in atelectasis.
22.2.1.4 PEEP
Adjusting PEEP is the primary means of controlling mean airway pressure and therefore oxygenation. Because the I:E ratio is typically 1:6, meaning that the bulk of each “respiratory cycle” is at the level of PEEP, airway pressure is only modestly above the level of PEEP. Consequently, PEEP values necessary for adequate mean airway pressures are higher than those used with conventional ventilation. Put another way, when changing from conventional ventilation with a typical I:E ratio of 1:2, if PIP and PEEP are kept unchanged, the mean airway pressure will drop substantially. This may be somewhat desirable when treating air leak, but when treating atelectasis-prone lungs, this will result in progressive atelectasis. Consequently, PEEP should rarely be <6 cm H2O and PEEP values of 10–12 cm H2O are not unusual in infants with severe lung disease.
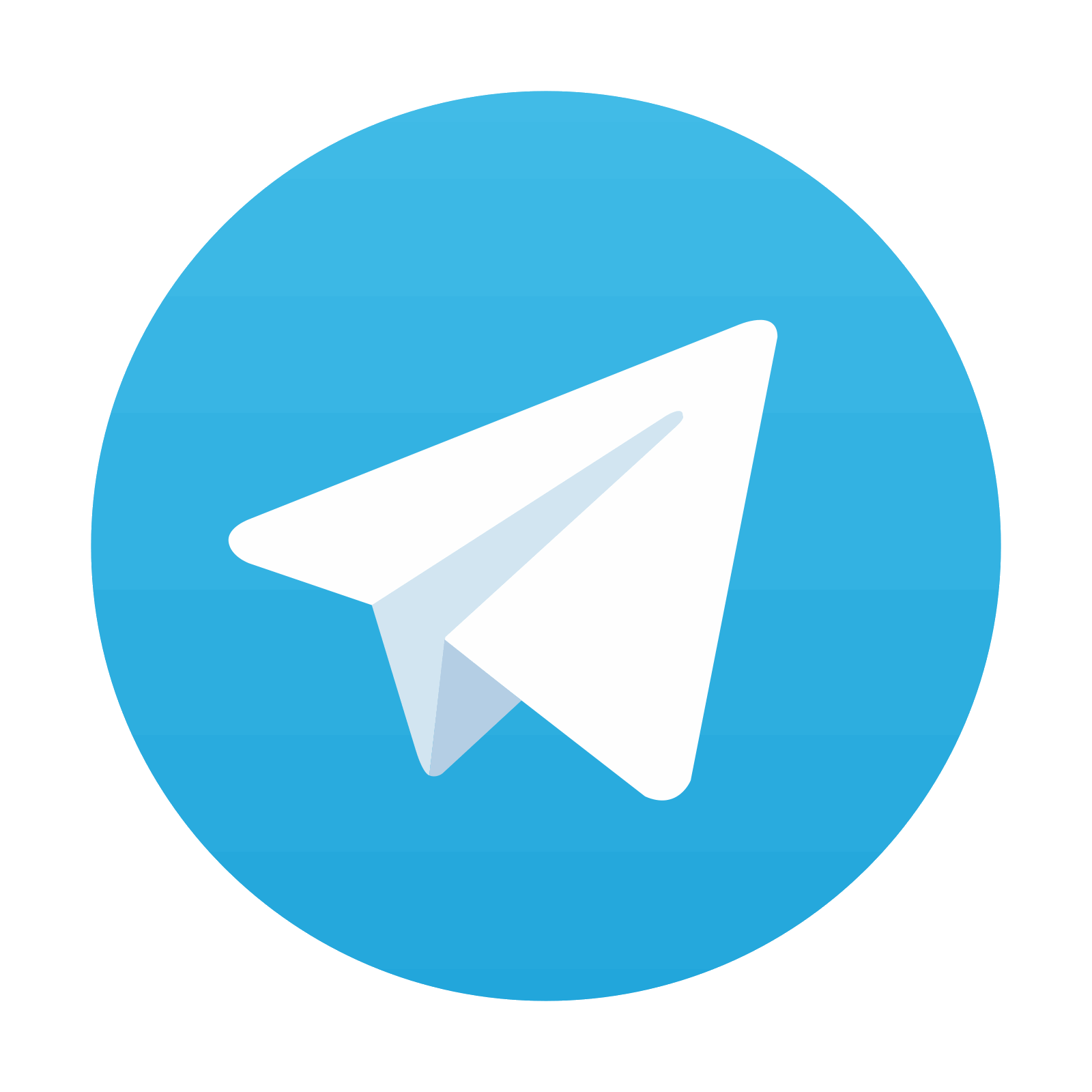
Stay updated, free articles. Join our Telegram channel
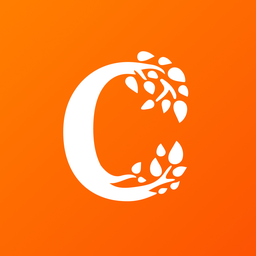
Full access? Get Clinical Tree
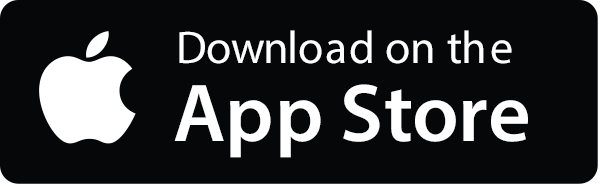
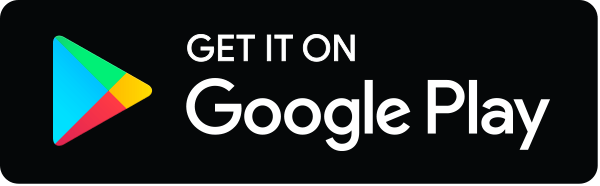